
An official website of the United States government
The .gov means it’s official. Federal government websites often end in .gov or .mil. Before sharing sensitive information, make sure you’re on a federal government site.
The site is secure. The https:// ensures that you are connecting to the official website and that any information you provide is encrypted and transmitted securely.
- Publications
- Account settings
- My Bibliography
- Collections
- Citation manager

Save citation to file
Email citation, add to collections.
- Create a new collection
- Add to an existing collection
Add to My Bibliography
Your saved search, create a file for external citation management software, your rss feed.
- Search in PubMed
- Search in NLM Catalog
- Add to Search
Cross Talk Between Metabolism and the Cell Division Cycle
Affiliations.
- 1 Cell Division and Cancer group, Spanish National Cancer Research Center (CNIO), Madrid, Spain.
- 2 Cell Division and Cancer group, Spanish National Cancer Research Center (CNIO), Madrid, Spain. [email protected].
- 3 Cancer Cell Cycle group, Vall d'Hebron Institute of Oncology (VHIO), Barcelona, Spain. [email protected].
- 4 ICREA, Barcelona, Spain. [email protected].
- PMID: 38393474
- DOI: 10.1007/978-1-0716-3557-5_9
Cell division requires a massive rewiring of cellular pathways, including molecular routes involved in providing energy for cell survival and functionality. The energetic requirements and the metabolic opportunities for generating energy change during the different phases of the cell cycle and how these processes are connected is still poorly understood. This chapter discusses basic concepts for a coordinated analysis of cell cycle progression and metabolism and provides specific protocols for studying these two connected processes in mammalian cells.
Keywords: Cell cycle; Cell synchronization; Cellular energy; Metabolism.
© 2024. The Author(s), under exclusive license to Springer Science+Business Media, LLC, part of Springer Nature.
PubMed Disclaimer
Similar articles
- Synchronization of Green Algae by Light and Dark Regimes for Cell Cycle and Cell Division Studies. Hlavová M, Vítová M, Bišová K. Hlavová M, et al. Methods Mol Biol. 2016;1370:3-16. doi: 10.1007/978-1-4939-3142-2_1. Methods Mol Biol. 2016. PMID: 26659950
- Strengths and Weaknesses of Cell Synchronization Protocols Based on Inhibition of DNA Synthesis. Ligasová A, Koberna K. Ligasová A, et al. Int J Mol Sci. 2021 Oct 5;22(19):10759. doi: 10.3390/ijms221910759. Int J Mol Sci. 2021. PMID: 34639098 Free PMC article. Review.
- How yeast coordinates metabolism, growth and division. Ewald JC. Ewald JC. Curr Opin Microbiol. 2018 Oct;45:1-7. doi: 10.1016/j.mib.2017.12.012. Epub 2018 Jan 13. Curr Opin Microbiol. 2018. PMID: 29334655 Review.
- Coordination of cell growth and cell division: a mathematical modeling study. Qu Z, Weiss JN, MacLellan WR. Qu Z, et al. J Cell Sci. 2004 Aug 15;117(Pt 18):4199-207. doi: 10.1242/jcs.01294. Epub 2004 Jul 27. J Cell Sci. 2004. PMID: 15280433
- A possible cross-talk of retinoic acid- and TPA-driven myeloid differentiation pathways. Zemanová K, Smarda J. Zemanová K, et al. Folia Biol (Praha). 1998;44(3):97-105. Folia Biol (Praha). 1998. PMID: 10730850
- Morgan DO (2006) The cell cycle: principles of control (primers in biology). Sinauer Associates, Inc
- Salazar-Roa M, Malumbres M (2017) Fueling the cell division cycle. Trends Cell Biol 27(1):69–81. https://doi.org/10.1016/j.tcb.2016.08.009 - DOI - PubMed
- Takhaveev V, Ozsezen S, Smith EN, Zylstra A, Chaillet ML, Chen H, Papagiannakis A, Milias-Argeitis A, Heinemann M (2023) Temporal segregation of biosynthetic processes is responsible for metabolic oscillations during the budding yeast cell cycle. Nat Metab 5(2):294–313. https://doi.org/10.1038/s42255-023-00741-x - DOI - PubMed - PMC
- Fajas L (2013) Re-thinking cell cycle regulators: the cross-talk with metabolism. Front Oncol 3:4. https://doi.org/10.3389/fonc.2013.00004 - DOI - PubMed - PMC
- Solaki M, Ewald JC (2018) Fueling the cycle: CDKs in carbon and energy metabolism. Front Cell Dev Biol 6:93. https://doi.org/10.3389/fcell.2018.00093 - DOI - PubMed - PMC
- Search in MeSH
Related information
Linkout - more resources, full text sources.
- Citation Manager
NCBI Literature Resources
MeSH PMC Bookshelf Disclaimer
The PubMed wordmark and PubMed logo are registered trademarks of the U.S. Department of Health and Human Services (HHS). Unauthorized use of these marks is strictly prohibited.
Cell division paper, Education

- Visva Bharati University
Abstract and Figures

Discover the world's research
- 25+ million members
- 160+ million publication pages
- 2.3+ billion citations
- Iin Murtini

- Elif Küçükkeskin

- Bhian Ananda Javanica Rubiyanto
- Puguh Karyanto

- N. González

- Bakhtaver S. Mahajan

- Homi Bhabha

- Fulya Öztap

- M. H. Hansell
- Chris R. Brown
- Jenny Lewis
- Colin Wood-Robinson
- Recruit researchers
- Join for free
- Login Email Tip: Most researchers use their institutional email address as their ResearchGate login Password Forgot password? Keep me logged in Log in or Continue with Google Welcome back! Please log in. Email · Hint Tip: Most researchers use their institutional email address as their ResearchGate login Password Forgot password? Keep me logged in Log in or Continue with Google No account? Sign up

An official website of the United States government
The .gov means it’s official. Federal government websites often end in .gov or .mil. Before sharing sensitive information, make sure you’re on a federal government site.
The site is secure. The https:// ensures that you are connecting to the official website and that any information you provide is encrypted and transmitted securely.
- Publications
- Account settings
Preview improvements coming to the PMC website in October 2024. Learn More or Try it out now .
- Advanced Search
- Journal List
- Cold Spring Harb Perspect Biol
- v.7(5); 2015 May
Meiosis: An Overview of Key Differences from Mitosis
Meiosis is the specialized cell division that generates gametes. In contrast to mitosis, molecular mechanisms and regulation of meiosis are much less understood. Meiosis shares mechanisms and regulation with mitosis in many aspects, but also has critical differences from mitosis. This review highlights these differences between meiosis and mitosis. Recent studies using various model systems revealed differences in a surprisingly wide range of aspects, including cell-cycle regulation, recombination, postrecombination events, spindle assembly, chromosome–spindle interaction, and chromosome segregation. Although a great degree of diversity can be found among organisms, meiosis-specific processes, and regulation are generally conserved.
Cells undergoing meiosis are unique in many respects (e.g., suppression of the S phase during the second round of cell division). The molecular mechanisms that underlie these critical events are beginning to be understood.
Meiosis is a special mode of cell division, which makes haploid cells from a diploid cell. It is essential for sexual reproduction in eukaryotes and diploid organisms and produces gametes, such as eggs and sperm. Sexual reproduction is thought to be essential for long-term survival of species, as it generates diversity and mixes the genetic materials within the species. This consists of two opposite processes: meiosis, which reduces chromosome numbers from diploid to haploid, and conjugation (fertilization), which restores the diploid state by fusion of two haploid cells. Meiosis generates diversity through two events: recombination and chromosome segregation. Missegregation during meiosis results in aneuploidy in progeny or fertilized eggs. In the case of humans, it is reported that 20% of all eggs are aneuploids, most of which are results of chromosome missegregation in oocytes ( Hassold and Hunt 2001 ). This is a major cause of infertility, miscarriages, and birth defects, such as Down’s syndrome, in humans. Despite the medical importance, little is known about the molecular mechanisms of meiotic chromosome segregation in humans. Understanding meiosis is not only important for its own ends, but also provides unique insights into the fundamental regulation of mitosis. As many excellent reviews already cover specific aspects of meiosis, this review gives an overview by highlighting key meiotic events and molecular regulation distinct from mitosis.
CELL-CYCLE CONTROL
In eukaryotic mitotic cycles, chromosome replication and segregation alternate. This is essential for maintaining the genome stability. This is achieved by two-step regulation of replication by Cdk ( Tanaka and Araki 2010 ). The first step, called licensing, allows Mcm2–7 to be recruited to form the prereplicative complex at replication origins only in G 1 when Cdk activity is low. An increase in Cdk kinase activity, together with Cdc7 kinase activity in late G 1 , triggers initiation of DNA replication. As a high Cdk activity inhibits the formation of the prereplicative complex, the origin will not be licensed until the mitotic exit. This dual function of Cdk ensures only one firing from each replication fork in one mitotic cycle.
In contrast, meiosis consists of two divisions without an intervening S phase, which is essential for reducing the ploidy. Suppression of the intervening S phase is achieved by maintaining the Cdk activity sufficiently high between two meiotic divisions. In Xenopus oocytes, incomplete degradation of cyclin B and a low amount of the Wee1 kinase keeps the Cdk activity high. Artificial inactivation of Cdk1 after meiosis I results in DNA replication between the two divisions ( Furuno et al. 1994 ; Iwabuchi et al. 2000 ; Nakajo et al. 2000 ). High Cdk1 activity inhibits the formation of the prereplicative complex by preventing binding of Mcm2–7 to replication origins. In Saccharomyces cerevisiae , a meiosis-specific protein kinase, Ime2, also contributes to phosphorylation of some of Cdk1 substrates to suppress replication between the two meiotic divisions ( Holt et al. 2007 ).
PAIRING AND RECOMBINATION
Meiotic recombination exchanges the genetic materials between two homologous chromosomes. It is essential not only for exchanging genetic materials to generate diversity in offspring, but also for holding homologous chromosomes together through chiasma, to segregate chromosomes properly.
Homologous chromosomes pair along the whole length and this homologous paring is further stabilized by the formation of an elaborate structure, the synaptonemal complex. In yeast and mouse, meiotic recombination is required for proper synaptonemal complex formation ( Loidl et al. 1994 ; Baudat et al. 2000 ; Romanienko and Camerini-Otero 2000 ), whereas in Drosophila and Caenorhabditis elegans , the synaptonemal complex can form independently of meiotic recombination ( Dernburg et al. 1998 ; McKim et al. 1998 ).
Recombination mechanisms themselves are largely shared in both meiotic recombination and the homologous recombination repair process in the mitotic cell cycle, but there are crucial differences. In the case of meiosis, DNA double-strand breaks (DSBs) are obligatory rather than the result of accidental damage, as in the mitotic cell cycle. DSBs, which initiate meiotic recombination, are created by the conserved Spo11 endonuclease ( Keeney et al. 1997 ). The sites of DSBs are not random, often clustering at meiotic recombination hot spots ( Lichten and Goldman 1995 ). There is a line of evidence that the chromatin modifications are involved in the site selection of meiotic DSBs. In S. cerevisiae , methylation of histone 3 at lysine 4 (H3K4) coincides with sites of DSBs, and the H3K4 methyltransferase Set1 is required for DSB formation ( Sollier et al. 2004 ; Borde et al. 2009 ). In mammalians, Prdm9, a H3K4 methyltransferase with a zinc finger domain, mediates the hot spot selection. The difference in the choice of hot spots among mouse strains was attributed to a difference in the amino acid sequence within the zinc finger domain ( Baudat et al. 2010 ; Parvanov et al. 2010 ). In humans, the major Prdm9 isoform within the population was predicted and shown to specifically bind the known consensus sequence enriched near recombination hot spots ( Baudat et al. 2010 ; Meyer et al. 2010 ). Furthermore, the allelic differences in the zinc finger domain are correlated to the usages of recombination hot spots in humans. Prdm9 is a fast-evolving protein in many animals, including humans ( Oliver et al. 2010 ), and this rapid change is thought to counteract a loss of individual hot spots because of biased gene conversion during the recombination process ( Nicolas et al. 1989 ).
The second difference is that the recombination partners are mainly homologous chromosomes in meiosis, whereas they are mainly sister chromatids in DNA repair during mitotic cycles ( Kadyk and Hartwell 1992 ; Bzymek et al. 2010 ). This homolog bias in meiosis is crucial as recombination among sister chromatids would not be productive in terms of generating diversity or forming the chiasma that hold homologous chromosomes together during metaphase I. From studies in S. cerevisiae , the partner choice is thought to be mediated by strand exchange proteins (RecA homologs), Rad51 and Dmc1, which promote the invasion of single-stranded DNA into a double-stranded recombination partner. Rad51 is expressed both in mitotic cycles and meiosis and, on its own, promotes intersister chromatid recombination, whereas the meiosis-specific protein Dmc1, together with Rad51, promotes interhomolog recombination in meiosis ( Cloud et al. 2012 ). In addition, interhomolog recombination is promoted in meiosis through suppression of Rad51 by two meiosis-specific factors: the kinase complex Red1/Hop1/Mek1 and the Rad51-interacting protein Hed1 ( Busygina et al. 2008 ; Niu et al. 2009 ).
During recombination, a specific arrangement of chromosomes, called a bouquet, has been observed in a wide variety of organisms ( Harper et al. 2004 ). In the bouquet arrangement, telomeres are attached to a specific area of the nuclear envelope. In the most well-studied organism, the fission yeast Schizosaccharomyces pombe , bouquet arrangement was shown to be associated with dynamic movement of the nucleus, which facilitates pairing and recombination ( Fig. 1 ) ( Chikashige et al. 1994 ). During fission yeast interphase, the spindle pole body (SPB) is associated with centromeres ( Funabiki et al. 1993 ). At the onset of meiosis, the SPB switches its association from centromeres to telomeres ( Chikashige et al. 1994 ). SUN and KASH domain proteins, together with Bqt1 and Bqt2, connect telomeres and cytoplasmic aster microtubules, which are organized by the meiosis-specific SPB protein Hrs1/Mcp6 ( Saito et al. 2005 ; Tanaka et al. 2005 ; Chikashige et al. 2006 ). The dynein motor drives the oscillatory movement of the nucleus to facilitate homologous chromosome pairing ( Yamamoto et al. 1999 ).

Bouquet formation and oscillatory nuclear movement in fission yeast meiosis. Clustering of telomeres and their linkage to the cytoskeleton enable oscillatory movement of the prophase nucleus and facilitate paring of homologous chromosomes. SPB, spindle pole body.
In C. elegans , the paring center near a telomere on each chromosome acts as the initiator of meiotic chromosome paring, and these pairing centers also interact with cytoplasmic dynein through links of SUN–KASH domain proteins, which span the nuclear envelope ( Sato et al. 2009 ; Baudrimont et al. 2010 ; Wynne et al. 2012 ). Movement along the nuclear envelope, mediated by dynein, induces dynamic movement of pairing centers. In mouse spermatocytes, bouquet organization and microtubule-dependent nuclear movement were reported during early meiotic prophase ( Scherthan et al. 1996 ; Morimoto et al. 2012 ). In addition, involvement of SUN–KASH domain proteins has been shown ( Morimoto et al. 2012 ).
An interesting example is found in S. cerevisiae . Vigorous chromosome movement is observed in meiotic prophase I ( Conrad et al. 2008 ; Koszul et al. 2008 ). Like other organisms, this chromosome movement is led by telomere cluster near spindle pole bodies, and a SUN domain protein is involved in this movement ( Rao et al. 2011 ). Surprisingly, actin filaments, not microtubules, are responsible for this movement ( Koszul et al. 2008 ).
POSTRECOMBINATION EVENTS
Compared with recombination and chromosome segregation, much less attention has been paid to the period between the two events in meiosis. However, this period is usually longest in meiosis. All mammalian oocytes arrest meiosis at birth until ovulation. This means that in human oocytes, arrest lasts up to 40 years. This prolonged arrest is linked to so-called maternal age effect in humans ( Hassold and Hunt 2009 ). Maternal age effect is the phenomenon that the incidence of aneuploidy increases as the age of the mothers increases. The cause is still under intense discussion, but cohesin fatigue is one of the attractive hypotheses. In mitotic cycles, cohesin establishes at S phase and the same cohesin complex stays on chromosomes until mitosis ( Uhlmann and Nasmyth 1998 ). If there is no new cohesin loading during the meiotic arrest, the same cohesin molecules have to keep chromatids together for decades. It is hypothesized that gradual loss of cohesin during the prolonged arrest probably increases the frequency of missegregation. Evidences suggest that cohesin does not turn over in mouse oocytes once it is established ( Revenkova et al. 2010 ; Tachibana-Konwalski et al. 2010 ), and oocytes from old mothers have reduced cohesin on chromosomes in mouse ( Lister et al. 2010 ).
During the postrecombination period, in some species, a compact cluster of chromosomes forms in the enlarged nucleus. In Drosophila oocytes, the structure was called the karyosome and forms soon after the completion of recombination ( King 1970 ). Similar clustering of chromatin within the nucleus can be found within mammalian oocytes. In mouse oocytes, two types of chromatin organization were found in immature oocytes, which are often referred to as SN (surrounded nucleolus) and NSN (nonsurrounded nucleolus). In a nucleus with SN, meiotic chromosomes are clustered around the nucleolus with centromeres in proximity to the nucleolus. This clustered chromatin is also referred to as the karyosphere, and is also found in human oocytes ( Parfenov et al. 1989 ). In mouse, oocytes with an SN configuration are more competent for further development after fertilization than ones with an NSN configuration ( Zuccotti et al. 1998 , 2002 ). From studies in Drosophila , it is proposed that clustering of meiotic chromosomes facilitates formation of one unified spindle ( Lancaster et al. 2007 ). As oocytes have a large nucleus and cytoplasm and spindles assembled around chromosomes without centrosomes, chromosomes distant from each other may form separate spindles. Although chromosome clustering is a widespread phenomenon in oocytes, very few molecular studies have, so far, been performed on the molecular basis of this process. In Drosophila oocytes, karyosome formation requires the conserved kinase NHK-1 ( Cullen et al. 2005 ; Ivanovska et al. 2005 ). A study identified barrier-to-autointegration factor (BAF), a linker protein between chromosomes and the nuclear envelope, as one of the critical substrates of NHK-1 in meiosis ( Fig. 2 ) ( Lancaster et al. 2007 ). It is proposed that the phosphorylation of BAF by NHK-1 is required for release of chromatin from the nuclear envelope to allow the karyosome formation. A further study showed that NHK-1 activity is suppressed by the meiotic recombination checkpoint to block nuclear reorganization, including karyosome formation, in response to unrepaired DSBs ( Lancaster et al. 2010 ).

Formation of the karyosome in Drosophila oocytes. The conserved protein kinase NHK-1 phosphorylates barrier-to-autointegration factor (BAF), a linker between the nuclear envelope and chromatin, to release meiotic chromosomes from the nuclear envelope. The meiotic recombination checkpoint suppresses NHK-1 activity to keep the nucleus in the recombination state when DNA double-strand breaks (DSBs) are still present.
REDUCTIONAL AND EQUATIONAL CHROMOSOME SEGREGATION
Homologous chromosomes are segregated in the first meiotic division, and sister chromatids are segregated in the second division. To achieve this, two major processes are specifically modified in meiosis in comparison with mitosis ( Fig. 3 ).

Reductional and equational chromosome segregation. Cohesin connects sister chromatids. In mitosis, sister kinetochores are attached to microtubules from the opposite poles. Cohesin connects sister chromatids and the removal of cohesin along chromosomes triggers sister chromatid separation. Homologous chromosomes behave independently. In meiosis I, sister kinetochores are attached to microtubules from the same pole. Homologous chromosomes are attached to the opposite poles and connected by chiasma. Destruction of cohesin from chromosome arms triggers homologous chromosome separation. Cohesin at centromeres is protected to provide a linkage among sister chromatids. In meiosis II, sister kinetochores are attached to microtubules from the opposite poles. Destruction of the centromeric cohesin triggers sister chromatid separation.
Monopolar Attachment of Sister Chromatids in Meiosis I
The first difference of meiosis from mitosis is the behavior of kinetochores to achieve bipolar attachment. In mitosis, sister kinetochores must attach to the opposite poles. In contrast, in meiosis I, sister kinetochores must attach to the same pole and homologous kinetochores must attach to the opposite poles. This is the key division that reduces the ploidy of cells in meiosis. In meiosis II, like mitosis, sister kinetochores must attach to the opposite poles.
In S. cerevisiae , the monopolin complex is responsible for monopolar orientation of sister kinetochores in meiosis I ( Tóth et al. 2000 ; Rabitsch et al. 2003 ). The monopolin complex consists of casein kinase I and other regulatory subunits, and localizes to kinetochores in meiosis ( Petronczki et al. 2006 ). Monopolin localization is dependent on Spo13, Polo kinase, and Cdc7 kinase ( Clyne et al. 2003 ; Katis et al. 2004 ; Lee et al. 2004 ; Lo et al. 2008 ; Matos et al. 2008 ).
However, the involvement of the monopolin complex in monoorientation of sister kinetochores may be restricted to S. cerevisiae , which has a single microtubule attached to each kinetochore. In fission yeast, in which multiple microtubules attach to each kinetochore, the homologous complex of monopolin is required for preventing one kinetochore from attaching microtubules from opposite poles (so-called merotelic attachment) in mitosis ( Gregan et al. 2007 ). Therefore, it is hypothesized that the molecular function of monopolin is to clamp microtubule attachment sites together between two sister kinetochores in the case of S. cerevisiae , and within a single kinetochore in mitosis in other organisms, which have multiple microtubules attached to one kinetochore ( Gregan et al. 2007 ; Corbett et al. 2010 ). Instead, in fission yeast, mono-orientation of sister kinetochores is dependent on the meiosis-specific cohesin subunit Rec8, as well as the meiosis-specific protein Moa1, which localizes to kinetochores until metaphase I ( Watanabe et al. 2001 ; Yokobayashi and Watanabe 2005 ). Rec8 and Moa1 interact with each other, but the molecular function of Moa1 remains to be understood. During meiosis I, the ability of the meiotic cohesin complex–containing Rec8 to localize at the core centromeres is necessary to promote mono-orientation of sister kinetochores, whereas the mitotic cohesin localizes at pericentrometic regions, not core centromeres, to promote biorientation of sister kinetochores ( Sakuno et al. 2009 ). Therefore, it is hypothesized that linking two sister centromeres by cohesin brings meiosis I–specific kinetochore configuration.
Stepwise Removal of Cohesin
The second difference of meiosis from mitosis is stepwise removal of cohesin from chromosomes. Cohesin connects sister chromatids consisting of replicated DNA ( Nasmyth and Haering 2009 ). In mitotic metaphase, cohesin resists the pulling forces acting on kinetochores toward the opposite poles. The cohesin complex is removed either by phosphorylation or cleavage of one of the subunits, Scc1. This removal triggers the separation of sister chromatids.
In contrast, in meiosis I, homologous chromosomes are connected by chiasma and pulled toward the opposite poles. This connection depends on cohesin localized among the sister chromatids distal to the chiasma. Removal of cohesin from chromosome arms abolishes the connection and triggers anaphase. The crucial difference from mitosis is that cohesin at centromeres must be protected in the metaphase/anaphase transition in meiosis I. This centromeric cohesin maintains a link among sister chromatids until anaphase II, when the remaining cohesin is removed. In most organisms, the meiosis-specific cohesin subunit Rec8 replaces Scc1 ( Watanabe and Nurse 1999 ). A conserved protein, called Shugoshin (Sgo), is responsible for this centromere protection ( Kitajima et al. 2004 ). Mei-S332 in Drosophila was the first identified member of Sgo. Mutants in mei-S332 showed precocious separation of sister chromatids in anaphase I, leading to missegregation of sister chromatid in the second meiotic division ( Kerrebrock et al. 1992 ). Later, this was shown to be widely conserved in eukaryotes when a homolog of mei-S332, Sgo1, was identified in fission yeast as a protein that protects the meiotic cohesin subunit Rec8 from proteolysis in the centromeric regions in anaphase I ( Kitajima et al. 2004 ). Both Rec8 and Sgo1 are expressed only in meiosis, and forced expression of both proteins in mitotic cells blocks nuclear division. Sgo recruits protein phosphatase 2A (PP2A) to centromeric regions and constantly dephosphorylates cohesin ( Kitajima et al. 2006 ; Riedel et al. 2006 ). As phosphorylation of cohesin is required for cleavage, Sgo protects meiotic cohesin from cleavage in anaphase I. Sgo itself is recruited to centromeres by phosphorylation of histone 2A by Bub1 kinase ( Kawashima et al. 2010 ).
In addition to the roles in meiosis, Sgo also has roles in ensuring the accuracy of chromosome segregation in mitosis ( Yao and Dai 2012 ). Although the molecular mechanism is still under investigation, evidence showed that it recruits and regulates various proteins at centromeres, including PP2A, the chromosomal passenger complex (CPC), and the microtubule-depolymerizing kinesin MCAK ( Tanno et al. 2010 ; Rivera et al. 2012 ). The identification and subsequent studies of Sgo are a good example of how the studies of meiosis have made crucial contributions to the understanding of mitosis.
ACENTROSOMAL SPINDLE FORMATION
A spindle in oocytes differs from a mitotic spindle in some key aspects. Remarkably, a spindle forms without centrosomes in the oocytes of many animals, including humans, mouse, Xenopus , Drosophila , and C. elegans ( McKim and Hawley 1995 ). This is specific to the oocyte not meiosis in general, as spermatocytes still contain centrosomes that drive spindle formation. Centrosomes must be eliminated or inactivated during oogenesis, but the mechanism of this is not understood. Lack of centrosomes in oocytes raises a question as to how spindle microtubules are assembled. An in vitro spindle-assembly system in Xenopus extract played critical roles in solving the problem. It was shown that beads coated with random DNA can assemble a bipolar spindle in Xenopus extract ( Heald et al. 1996 ). This indicates any DNA can recruit proteins that induce microtubule assembly. It revealed the central role of the Ran system in chromatin-mediated assembly of spindle microtubules ( Gruss et al. 2001 ; Wiese et al. 2001 ). The Ran system was originally identified for nuclear transport, but, subsequently, identified for spindle assembly and nuclear envelope reassembly ( Hetzer et al. 2002 ). Ran is a small G protein that can be switched between GTP- and GDP-binding forms ( Fig. 4 ). A chromosome-associated protein, Rcc1, acts as a guanine nucleotide-exchanging factor (GEF), which converts Ran-GDP to Ran-GTP to generate the Ran-GTP gradient. Ran-GTP binds to importin by removing it from other binding proteins, including some “spindle-assembly factors,” which promote spindle assembly. Away from the chromosomes in which the Ran-GDP form is dominating, these spindle-assembly factors are kept inactive by binding to importin. Near the chromosomes in which Ran-GTP concentration is high, the spindle-assembly factors are released from importin to become active. These spindle-assembly factors include TPX2, NuMA, NuSAP, and HURP, and these collectively promote microtubule stabilization and bipolar spindle formation ( Gruss et al. 2001 ; Wiese et al. 2001 ; Koffa et al. 2006 ; Ribbeck et al. 2006 ; Sillje et al. 2006 ).

Chromosome-mediated spindle assembly through Ran. Ran-GDP is converted into Ran-GTP by chromosome-associated RCC1 to generate a Ran-GTP gradient. Near chromosomes, Ran-GTP activates spindle-assembly factors by removing importin from them. Microtubules and the spindle can be assembled only in proximity to the chromosomes.
The requirement of the Ran-GTP gradient-based mechanisms in chromosome-mediated acentrosomal spindle assembly is very clear in Xenopus extract, but it is less clear in living oocytes. Disrupting the Ran gradient by either expression of dominant negative or hyperactive forms of Ran did not prevent a spindle from forming around the chromosomes in mouse oocytes ( Dumont et al. 2007 ). Similar observations have been made in Drosophila oocytes, although the gradient was not directly monitored ( Cesario and McKim 2011 ). This indicates that chromosomes have alternative pathways or signals that induce microtubule assembly independently from Ran. The CPC-containing Aurora B kinase may act as an alternative pathway. First, in Xenopus egg extract, the CPC is essential for centrosome-independent spindle microtubule assembly ( Sampath et al. 2004 ). Also, in Drosophila oocytes, it was shown that CPC is essential for spindle microtubule assembly ( Colombie et al. 2008 ; Radford et al. 2012 ). Chromosomes activate Aurora B kinase independently of Ran and the activated kinase is then targeted to microtubules to promote spindle assembly ( Tseng et al. 2010 ). The targets of the kinase activity include two microtubule-depolymerizing proteins, MCAK and Op18/stathmin ( Andrews et al. 2004 ; Ohi et al. 2004 ; Gadea and Ruderman 2006 ).
Although it was known that the mitotic spindle forms without centrosomes in plants, it was, relatively, recently realized that the spindle can form in mitotic animal cells without centrosomes when they are artificially removed. In human cultured cells, when centrosomes were ablated using a laser, the spindle morphology and function were unaffected ( Khodjakov et al. 2000 ). In Drosophila , inactivating essential centrosome components eliminated centrosomes from cells, but spindle formation and function is not disrupted ( Basto et al. 2006 ). Furthermore, the flies lacking centrosomes develop with only a slight increase in the frequency of aneuploids.
As a mitotic spindle can form without centrosomes, a critical question is whether a meiotic spindle is simply the same as a mitotic spindle without centrosomes, or a spindle that is modified to cope with a lack of centrosomes? This is an unexplored question, but some evidence suggests the existence of oocyte-specific mechanisms to compensate for a lack of centrosomes. For example, in Drosophila mitosis, the γ-tubulin recruiting complex augmin is responsible for assembling most centrosome-independent spindle microtubules ( Goshima et al. 2008 ). Therefore, a loss of the augmin complex, in conjunction with inactivation of centrosomes, results in a dramatic loss of spindle microtubules ( Goshima et al. 2008 ; Wainman et al. 2009 ). In contrast, oocytes lacking the augmin complex (and centrosomes) still form robust spindles ( Meireles et al. 2009 ). This suggests a meiosis-specific microtubule assembly pathway independent of centrosomes and augmin. Moreover, augmin shows meiosis-specific stable localization to acentrosomal spindle poles, suggesting that the meiosis-specific regulation of augmin may, in part, compensate for a lack of centrosomes in oocytes ( Colombie et al. 2013 ).
ASYMMETRIC DIVISION OF OOCYTES
Meiosis produces four daughter haploid cells from one diploid oocyte. In the case of oogenesis, only one daughter becomes an egg and the others (polar bodies) will not participate in reproduction. Oocytes divide asymmetrically in each division to minimize a loss of the cytoplasm. For successful asymmetric division, the spindle must be positioned near the cell cortex and oriented perpendicularly to the cell cortex. Considerable studies on asymmetric divisions have been performed in mitosis, highlighting the critical roles of centrosomes and interaction between aster microtubules and the cell cortex ( Knoblich 2010 ). Without centrosomes in oocytes, how does the meiotic spindle become oriented and positioned? Studies in mouse oocytes showed that instead of microtubules, the dynamic actin network plays a crucial role in the positioning of the meiotic spindle. The actin network in oocytes is formed by cooperative actions of the actin nucleators, Formin-2 (Fmn2), Spire 1, and Spire 2 ( Azoury et al. 2008 ; Schuh and Ellenberg 2008 ; Pfender et al. 2011 ). Rab18a-positive vesicles serve as nodes of the network to regulate the density and myosin IVb-dependent dynamics ( Holubcová et al. 2013 ). Transient destabilization of actin filaments caused by temporal degradation of Fmn2 is required for initial migration ( Azoury et al. 2011 ).
Similarly, the meiosis II spindle needs to be positioned near the cortex. In mouse meiosis II, this is maintained by a flow of actin away from the spindle along the cortex and toward the spindle from the other side of the oocyte ( Yi et al. 2011 ). This flow is driven by Arp2/3, N-WASP, and myosin II. A similar cytoplasmic flow was also observed in the late stage of the spindle migration in meiosis I.
INTERACTION BETWEEN THE SPINDLE AND CHROMOSOMES
Chromosome–microtubule interactions in oocytes may be “different” from those in mitosis. In mitosis, the main interaction is provided by kinetochores, which interact with dynamic microtubule ends. In the simplest model of mitosis, microtubules nucleated from centrosomes capture kinetochores and generate pulling forces (the “search and capture” model) ( Kirschner and Mitchison 1986 ). When sister kinetochores are attached to microtubules from the opposite poles, chromosomes becomes congressed to the metaphase plate. The pulling forces acting between kinetochores and the opposite poles are resisted by cohesion among sister chromatids, and destruction of cohesin at the onset of anaphase triggers the movement of sister chromatids toward the poles. Although kinetochores are also important in meiosis, nonkinetochore interactions seem more prominent in oocytes than in mitotic cells.
In mouse, it has been shown that kinetochore-microtubule end-on attachment is not properly established until well after chromosome congression at the spindle equator ( Brunet et al. 1999 ). Chromosomes move toward the spindle equator by sliding along the surface of the spindle without end-on attachment, leading to ring arrangement of chromosomes at the spindle equator ( Kitajima et al. 2011 ). This congression is followed by trial-and-error establishment of bipolar end-on attachment of homologous kinetochores at the spindle equator. Full stable end-on attachment will not be achieved until several hours after nuclear envelope breakdown, and the delay of end-on attachment in oocytes appears to be caused by slow gradual increase of Cdk1 activity ( Davydenko et al. 2013 ). An artificial premature increase of Cdk1 activity resulted in the premature establishment of attachment. As this also increased the lagging chromosomes in anaphase I, slow increase of Cdk1 activity is proposed to delay stable attachment until spindle bipolarity is established. It remains to be established how the chromosomes congress to the spindle equator without end-on microtubule attachment to kinetochores or how a gradual increase of Cdk delays the microtubule attachment to kinetochores.
Observations in C. elegans oocytes also indicated different contributions of kinetochores in meiosis to those in mitosis. First, microtubules appear to interact with chromosomes laterally during chromosome congression. This congression is at least partly mediated by the chromokinesin KLP-19, which localizes to the junction among the homologs ( Wignall and Villeneuve 2009 ). Furthermore, inactivation of kinetochores by RNA interference (RNAi) resulted in less tight congression and misorientation of chromosomes relative to the spindle axis. Surprisingly, chromosomes without active kinetochores can separate during anaphase at a speed comparable with the wild type ( Dumont et al. 2010 ). Anaphase chromosome movement seems to be driven by the elongation of spindle microtubules among separating homologous chromosomes. However, it should be noted that C. elegans centromeres are not restricted to small regions, as kinetochores are formed along proximal parts of chromosomes in meiosis ( Dumont et al. 2010 ).
How do the chromosomes move without end-on attachment in oocytes? Even in mitosis, there is evidence of such forces acting on chromosomes. Polar ejection forces act on chromosome arms and are involved in chromosome congression at the metaphase plate ( Rieder and Salmon 1994 ). When chromosomes were artificially cut, a chromosome fragment that lacked kinetochores moved toward the spindle equator ( Rieder et al. 1986 ). Chromokinesins play a part in polar ejection forces, but interaction of chromosome arms with growing microtubule plus ends can also generate such forces. In the case of Drosophila oocytes, the chromokinesin Nod is thought to generate polar ejection forces ( Theurkauf and Hawley 1992 ; Matthies et al. 1999 ). Nod is an immotile kinesin but can promote microtubule polymerization ( Cui et al. 2005 ). In mouse oocytes, the chromokinesin Kid is dispensable for chromosome congression ( Kitajima et al. 2011 ).
SPINDLE-ASSEMBLY CHECKPOINT
The spindle-assembly checkpoint is a mechanism to ensure the correct segregation of chromosomes and is crucial for genome stability. It monitors a lack of microtubule attachment to kinetochores and a lack of tension to block or delay anaphase onset through inhibition of anaphase-promoting complex/cyclosome (APC/C) ( Lara-Gonzalez et al. 2012 ).
There are lines of evidence that suggest that the spindle-assembly checkpoint in meiosis is not robust as in mitosis. This is evident especially in oocytes, which display a high incidence of chromosome missegregation. In mouse oocytes, several studies show that anaphase I can initiate without all chromosomes achieving proper bipolar attachment, metaphase alignment, or interkinetochore tension ( LeMaire-Adkins et al. 1997 ; Nagaoka et al. 2011 ; Kolano et al. 2012 ). In Xenopus oocytes, inhibition of spindle microtubules or bipolar spindle formation did not delay the onset of anaphase I ( Shao et al. 2013 ). This lack of a robust spindle checkpoint in oocytes may be one of the reasons why meiosis in human oocytes shows a high level of chromosome missegregation.
Although tension among homologous chromosomes, not sister chromatids, has to be detected in meiosis I, the molecular mechanism may be shared with mitosis. During meiotic prometaphase I in yeast and mouse oocytes, the CPC is essential for releasing incorrect attachments ( Kitajima et al. 2011 ; Meyer et al. 2013 ) to achieve the bipolar attachment of homologs. In Drosophila oocytes, it has been shown that the CPC is required for bipolar attachment ( Resnick et al. 2009 ). Therefore, the requirement of the CPC in correcting erroneous attachment is universal in mitosis and meiosis, and conserved among eukaryotes.
CONCLUDING REMARKS
The study of meiosis has a long history, but far fewer studies have been performed on meiosis in comparison with mitosis, partly because of technical challenges. Although studies of meiosis often generated results that are largely extensions of what is already known in mitosis, some studies have revealed unexpected functions and regulations of meiosis. In some cases, they had impacts well beyond meiosis, especially on the understanding of mitosis. Recent studies have resulted in many important findings, and many more exciting discoveries are still waiting to come.
ACKNOWLEDGMENTS
I thank Robin Beaven for valuable comments. H.O. holds a Wellcome Trust Senior Research Fellowship in Basic Biomedical Science.
Editors: Mitsuhiro Yanagida, Anthony A. Hyman, and Jonathon Pines
Additional Perspectives on Mitosis available at www.cshperspectives.org
- Andrews PD, Ovechkina Y, Morrice N, Wagenbach M, Duncan K, Wordeman L, Swedlow JR. 2004. Aurora B regulates MCAK at the mitotic centromere . Dev Cell 6 : 253–268. [ PubMed ] [ Google Scholar ]
- Azoury J, Lee KW, Georget V, Rassinier P, Leader B, Verlhac MH. 2008. Spindle positioning in mouse oocytes relies on a dynamic meshwork of actin filaments . Curr Biol 18 : 1514–1519. [ PubMed ] [ Google Scholar ]
- Azoury J, Lee KW, Georget V, Hikal P, Verlhac MH. 2011. Symmetry breaking in mouse oocytes requires transient F-actin meshwork destabilization . Development 138 : 2903–2908. [ PubMed ] [ Google Scholar ]
- Basto R, Lau J, Vinogradova T, Gardiol A, Woods CG, Khodjakov A, Raff JW. 2006. Flies without centrioles . Cell 125 : 1375–1386. [ PubMed ] [ Google Scholar ]
- Baudat F, Manova K, Yuen JP, Jasin M, Keeney S. 2000. Chromosome synapsis defects and sexually dimorphic meiotic progression in mice lacking Spo11 . Mol Cell 6 : 989–998. [ PubMed ] [ Google Scholar ]
- Baudat F, Buard J, Grey C, Fledel-Alon A, Ober C, Przeworski M, Coop G, de Massy B. 2010. PRDM9 is a major determinant of meiotic recombination hotspots in humans and mice . Science 327 : 836–840. [ PMC free article ] [ PubMed ] [ Google Scholar ]
- Baudrimont A, Penkner A, Woglar A, Machacek T, Wegrostek C, Gloggnitzer J, Fridkin A, Klein F, Gruenbaum Y, Pasierbek P, et al. 2010. Leptotene/zygotene chromosome movement via the SUN/KASH protein bridge in Caenorhabditis elegans . PLoS Genet 6 : e1001219. [ PMC free article ] [ PubMed ] [ Google Scholar ]
- Borde V, Robine N, Lin W, Bonfils S, Géli V, Nicolas A. 2009. Histone H3 lysine 4 trimethylation marks meiotic recombination initiation sites . EMBO J 28 : 99–111. [ PMC free article ] [ PubMed ] [ Google Scholar ]
- Brunet S, Maria AS, Guillaud P, Dujardin D, Kubiak JZ, Maro B. 1999. Kinetochore fibers are not involved in the formation of the first meiotic spindle in mouse oocytes, but control the exit from the first meiotic M phase . J Cell Biol 146 : 1–12. [ PMC free article ] [ PubMed ] [ Google Scholar ]
- Busygina V, Sehorn MG, Shi IY, Tsubouchi H, Roeder GS, Sung P. 2008. Hed1 regulates Rad51-mediated recombination via a novel mechanism . Genes Dev 22 : 786–795. [ PMC free article ] [ PubMed ] [ Google Scholar ]
- Bzymek M, Thayer NH, Oh SD, Kleckner N, Hunter N, 2010. Double Holliday junctions are intermediates of DNA break repair . Nature 464 : 937–941. [ PMC free article ] [ PubMed ] [ Google Scholar ]
- Cesario J, McKim KS. 2011. RanGTP is required for meiotic spindle organization and the initiation of embryonic development in Drosophila . J Cell Sci 124 : 3797–3810. [ PMC free article ] [ PubMed ] [ Google Scholar ]
- Chikashige Y, Ding DQ, Funabiki H, Haraguchi T, Mashiko S, Yanagida M, Hiraoka Y. 1994. Telomere-led premeiotic chromosome movement in fission yeast . Science 264 : 270–273. [ PubMed ] [ Google Scholar ]
- Chikashige Y, Tsutsumi C, Yamane M, Okamasa K, Haraguchi T, Hiraoka Y. 2006. Meiotic proteins bqt1 and bqt2 tether telomeres to form the bouquet arrangement of chromosomes . Cell 125 : 59–69. [ PubMed ] [ Google Scholar ]
- Cloud V, Chan YL, Grubb J, Budke B, Bishop DK. 2012. Rad51 is an accessory factor for Dmc1-mediated joint molecule formation during meiosis . Science 337 : 1222–1225. [ PMC free article ] [ PubMed ] [ Google Scholar ]
- Clyne RK, Katis VL, Jessop L, Benjamin KR, Herskowitz I, Lichten M, Nasmyth K. 2003. Polo-like kinase Cdc5 promotes chiasmata formation and cosegregation of sister centromeres at meiosis I . Nat Cell Biol 5 : 480–485. [ PubMed ] [ Google Scholar ]
- Colombié N, Cullen CF, Brittle AL, Jang JK, Earnshaw WC, Carmena M, McKim K, Ohkura H. 2008. Dual roles of Incenp crucial to the assembly of the acentrosomal metaphase spindle in female meiosis . Development 135 : 3239–3246. [ PMC free article ] [ PubMed ] [ Google Scholar ]
- Colombié N, Głuszek AA, Meireles AM, Ohkura H. 2013. Meiosis-specific stable binding of augmin to acentrosomal spindle poles promotes biased microtubule assembly in oocytes . PLoS Genet 9 : e1003562. [ PMC free article ] [ PubMed ] [ Google Scholar ]
- Conrad MN, Lee CY, Chao G, Shinohara M, Kosaka H, Shinohara A, Conchello JA, Dresser ME. 2008. Rapid telomere movement in meiotic prophase is promoted by NDJ1, MPS3, and CSM4 and is modulated by recombination . Cell 133 : 1175–1187. [ PubMed ] [ Google Scholar ]
- Corbett KD, Yip CK, Ee LS, Walz T, Amon A, Harrison SC. 2010. The monopolin complex crosslinks kinetochore components to regulate chromosome-microtubule attachments . Cell 142 : 556–567. [ PMC free article ] [ PubMed ] [ Google Scholar ]
- Cui W, Sproul LR, Gustafson SM, Matthies HJ, Gilbert SP, Hawley RS. 2005. Drosophila Nod protein binds preferentially to the plus ends of microtubules and promotes microtubule polymerization in vitro . Mol Biol Cell 16 : 5400–5409. [ PMC free article ] [ PubMed ] [ Google Scholar ]
- Cullen CF, Brittle AL, Ito T, Ohkura H. 2005. The conserved kinase NHK-1 is essential for mitotic progression and unifying acentrosomal meiotic spindles in Drosophila melanogaster . J Cell Biol 171 : 593–602. [ PMC free article ] [ PubMed ] [ Google Scholar ]
- Davydenko O, Schultz RM, Lampson MA. 2013. Increased CDK1 activity determines the timing of kinetochore-microtubule attachments in meiosis I . J Cell Biol 202 : 221–229. [ PMC free article ] [ PubMed ] [ Google Scholar ]
- Dernburg AF, McDonald K, Moulder G, Barstead R, Dresser M, Villeneuve AM. 1998. Meiotic recombination in C. elegans initiates by a conserved mechanism and is dispensable for homologous chromosome synapsis . Cell 94 : 387–398. [ PubMed ] [ Google Scholar ]
- Dumont J, Petri S, Pellegrin F, Terret ME, Bohnsack MT, Rassinier P, Georget V, Kalab P, Gruss OJ, Verlhac MH. 2007. A centriole- and RanGTP-independent spindle assembly pathway in meiosis I of vertebrate oocytes . J Cell Biol 176 : 295–305. [ PMC free article ] [ PubMed ] [ Google Scholar ]
- Dumont J, Oegema K, Desai A. 2010. A kinetochore-independent mechanism drives anaphase chromosome separation during acentrosomal meiosis . Nat Cell Biol 12 : 894–901. [ PMC free article ] [ PubMed ] [ Google Scholar ]
- Funabiki H, Hagan I, Uzawa S, Yanagida M. 1993. Cell cycle-dependent specific positioning and clustering of centromeres and telomeres in fission yeast . J Cell Biol 121 : 961–976. [ PMC free article ] [ PubMed ] [ Google Scholar ]
- Furuno N, Nishizawa M, Okazaki K, Tanaka H, Iwashita J, Nakajo N, Ogawa Y, Sagata N. 1994. Suppression of DNA replication via Mos function during meiotic divisions in Xenopus oocytes . EMBO J 13 : 2399–2410. [ PMC free article ] [ PubMed ] [ Google Scholar ]
- Gadea BB, Ruderman JV. 2006. Aurora B is required for mitotic chromatin-induced phosphorylation of Op18/Stathmin . Proc Natl Acad Sci 103 : 4493–4498. [ PMC free article ] [ PubMed ] [ Google Scholar ]
- Goshima G, Mayer M, Zhang N, Stuurman N, Vale RD. 2008. Augmin: A protein complex required for centrosome-independent microtubule generation within the spindle . J Cell Biol 181 : 421–429. [ PMC free article ] [ PubMed ] [ Google Scholar ]
- Gregan J, Riedel CG, Pidoux AL, Katou Y, Rumpf C, Schleiffer A, Kearsey SE, Shirahige K, Allshire RC, Nasmyth K. 2007. The kinetochore proteins Pcs1 and Mde4 and heterochromatin are required to prevent merotelic orientation . Curr Biol 17 : 1190–1200. [ PMC free article ] [ PubMed ] [ Google Scholar ]
- Gruss OJ, Carazo-Salas RE, Schatz CA, Guarguaglini G, Kast J, Wilm M, Le Bot N, Vernos I, Karsenti E, Mattaj IW. 2001. Ran induces spindle assembly by reversing the inhibitory effect of importin α on TPX2 activity . Cell 104 : 83–93. [ PubMed ] [ Google Scholar ]
- Harper L, Golubovskaya I, Cande WZ. 2004. A bouquet of chromosomes . J Cell Sci 117 : 4025–4032. [ PubMed ] [ Google Scholar ]
- Hassold T, Hunt P. 2001. To err (meiotically) is human: The genesis of human aneuploidy . Nat Rev Genet 2 : 280–291. [ PubMed ] [ Google Scholar ]
- Hassold T, Hunt P. 2009. Maternal age and chromosomally abnormal pregnancies: What we know and what we wish we knew . Curr Opin Pediatr 21 : 703–708. [ PMC free article ] [ PubMed ] [ Google Scholar ]
- Heald R, Tournebize R, Blank T, Sandaltzopoulos R, Becker P, Hyman A, Karsenti E. 1996. Self-organization of microtubules into bipolar spindles around artificial chromosomes in Xenopus egg extracts . Nature 382 : 420–425. [ PubMed ] [ Google Scholar ]
- Hetzer M, Gruss OJ, Mattaj IW. 2002. The Ran GTPase as a marker of chromosome position in spindle formation and nuclear envelope assembly . Nat Cell Biol 4 : E177–E184. [ PubMed ] [ Google Scholar ]
- Holt LJ, Hutti JE, Cantley LC, Morgan DO. 2007. Evolution of Ime2 phosphorylation sites on Cdk1 substrates provides a mechanism to limit the effects of the phosphatase Cdc14 in meiosis . Mol Cell 25 : 689–702. [ PMC free article ] [ PubMed ] [ Google Scholar ]
- Holubcová Z, Howard G, Schuh M. 2013. Vesicles modulate an actin network for asymmetric spindle positioning . Nat Cell Biol 15 : 937–947. [ PMC free article ] [ PubMed ] [ Google Scholar ]
- Ivanovska I, Khandan T, Ito T, Orr-Weaver TL. 2005. A histone code in meiosis: The histone kinase, NHK-1, is required for proper chromosomal architecture in Drosophila oocytes . Genes Dev 19 : 2571–2582. [ PMC free article ] [ PubMed ] [ Google Scholar ]
- Iwabuchi M, Ohsumi K, Yamamoto TM, Sawada W, Kishimoto T. 2000. Residual Cdc2 activity remaining at meiosis I exit is essential for meiotic M-M transition in Xenopus oocyte extracts . EMBO J 19 : 4513–4523. [ PMC free article ] [ PubMed ] [ Google Scholar ]
- Kadyk LC, Hartwell LH. 1992. Sister chromatids are preferred over homologs as substrates for recombinational repair in Saccharomyces cerevisiae . Genetics 132 : 387–402. [ PMC free article ] [ PubMed ] [ Google Scholar ]
- Katis VL, Matos J, Mori S, Shirahige K, Zachariae W, Nasmyth K. 2004. Spo13 facilitates monopolin recruitment to kinetochores and regulates maintenance of centromeric cohesion during yeast meiosis . Curr Biol 14 : 2183–2196. [ PubMed ] [ Google Scholar ]
- Kawashima SA, Yamagishi Y, Honda T, Ishiguro K, Watanabe Y. 2010. Phosphorylation of H2A by Bub1 prevents chromosomal instability through localizing shugoshin . Science 327 : 172–177. [ PubMed ] [ Google Scholar ]
- Keeney S, Giroux CN, Kleckner N. 1997. Meiosis-specific DNA double-strand breaks are catalyzed by Spo11, a member of a widely conserved protein family . Cell 88 : 375–384. [ PubMed ] [ Google Scholar ]
- Kerrebrock AW, Miyazaki WY, Birnby D, Orr-Weaver TL. 1992. The Drosophila mei-S332 gene promotes sister-chromatid cohesion in meiosis following kinetochore differentiation . Genetics 130 : 827–841. [ PMC free article ] [ PubMed ] [ Google Scholar ]
- Khodjakov A, Cole RW, Oakley BR, Rieder CL. 2000. Centrosome-independent mitotic spindle formation in vertebrates . Curr Biol 10 : 59–67. [ PubMed ] [ Google Scholar ]
- King RC. 1970. The meiotic behavior of the Drosophila oocyte . Int Rev Cytol 28 : 125–168. [ PubMed ] [ Google Scholar ]
- Kirschner M, Mitchison T. 1986. Beyond self-assembly: From microtubules to morphogenesis . Cell 45 : 329–342. [ PubMed ] [ Google Scholar ]
- Kitajima TS, Kawashima SA, Watanabe Y. 2004. The conserved kinetochore protein shugoshin protects centromeric cohesion during meiosis . Nature 427 : 510–517. [ PubMed ] [ Google Scholar ]
- Kitajima TS, Sakuno T, Ishiguro K, Iemura S, Natsume T, Kawashima SA, Watanabe Y. 2006. Shugoshin collaborates with protein phosphatase 2A to protect cohesin . Nature 441 : 46–52. [ PubMed ] [ Google Scholar ]
- Kitajima TS, Ohsugi M, Ellenberg J. 2011. Complete kinetochore tracking reveals error-prone homologous chromosome biorientation in mammalian oocytes . Cell 146 : 568–581. [ PubMed ] [ Google Scholar ]
- Knoblich JA. 2010. Asymmetric cell division: Recent developments and their implications for tumour biology . Nat Rev Mol Cell Biol 11 : 849–860. [ PMC free article ] [ PubMed ] [ Google Scholar ]
- Koffa MD, Casanova CM, Santarella R, Köcher T, Wilm M, Mattaj IW. 2006. HURP is part of a Ran-dependent complex involved in spindle formation . Curr Biol 16 : 743–754. [ PubMed ] [ Google Scholar ]
- Kolano A, Brunet S, Silk AD, Cleveland DW, Verlhac MH. 2012. Error-prone mammalian female meiosis from silencing the spindle assembly checkpoint without normal interkinetochore tension . Proc Natl Acad Sci 109 : E1858–E1867. [ PMC free article ] [ PubMed ] [ Google Scholar ]
- Koszul R, Kim KP, Prentiss M, Kleckner N, Kameoka S. 2008. Meiotic chromosomes move by linkage to dynamic actin cables with transduction of force through the nuclear envelope . Cell 133 : 1188–1201. [ PMC free article ] [ PubMed ] [ Google Scholar ]
- Lancaster OM, Cullen CF, Ohkura H. 2007. NHK-1 phosphorylates BAF to allow karyosome formation in the Drosophila oocyte nucleus . J Cell Biol 179 : 817–824. [ PMC free article ] [ PubMed ] [ Google Scholar ]
- Lancaster OM, Breuer M, Cullen CF, Ito T, Ohkura H. 2010. The meiotic recombination checkpoint suppresses NHK-1 kinase to prevent reorganisation of the oocyte nucleus in Drosophila . PLoS Genet 6 : e1001179. [ PMC free article ] [ PubMed ] [ Google Scholar ]
- Lara-Gonzalez P, Westhorpe FG, Taylor SS. 2012. The spindle assembly checkpoint . Curr Biol 22 : R966–R980. [ PubMed ] [ Google Scholar ]
- Lee BH, Kiburz BM, Amon A. 2004. Spo13 maintains centromeric cohesion and kinetochore coorientation during meiosis I . Curr Biol 14 : 2168–2182. [ PubMed ] [ Google Scholar ]
- LeMaire-Adkins R, Radke K, Hunt PA. 1997. Lack of checkpoint control at the metaphase/anaphase transition: A mechanism of meiotic nondisjunction in mammalian females . J Cell Biol 139 : 1611–1619. [ PMC free article ] [ PubMed ] [ Google Scholar ]
- Lichten M, Goldman AS. 1995. Meiotic recombination hotspots . Annu Rev Genet 29 : 423–444. [ PubMed ] [ Google Scholar ]
- Lister LM, Kouznetsova A, Hyslop LA, Kalleas D, Pace SL, Barel JC, Nathan A, Floros V, Adelfalk C, Watanabe Y, et al. 2010. Age-related meiotic segregation errors in mammalian oocytes are preceded by depletion of cohesin and Sgo2 . Curr Biol 20 : 1511–1521. [ PubMed ] [ Google Scholar ]
- Lo HC, Wan L, Rosebrock A, Futcher B, Hollingsworth NM. 2008. Cdc7-Dbf4 regulates NDT80 transcription as well as reductional segregation during budding yeast meiosis . Mol Biol Cell 19 : 4956–4967. [ PMC free article ] [ PubMed ] [ Google Scholar ]
- Loidl J, Klein F, Scherthan H. 1994. Homologous pairing is reduced but not abolished in asynaptic mutants of yeast . J Cell Biol 125 : 1191–1200. [ PMC free article ] [ PubMed ] [ Google Scholar ]
- Matos J, Lipp JJ, Bogdanova A, Guillot S, Okaz E, Junqueira M, Shevchenko A, Zachariae W. 2008. Dbf4-dependent CDC7 kinase links DNA replication to the segregation of homologous chromosomes in meiosis I . Cell 135 : 662–678. [ PubMed ] [ Google Scholar ]
- Matthies HJ, Messina LG, Namba R, Greer KJ, Walker MY, Hawley RS. 1999. Mutations in the α-tubulin 67C gene specifically impair achiasmate segregation in Drosophila melanogaster . J Cell Biol 147 : 1137–1144. [ PMC free article ] [ PubMed ] [ Google Scholar ]
- McKim KS, Hawley RS. 1995. Chromosomal control of meiotic cell division . Science 270 : 1595–1601. [ PubMed ] [ Google Scholar ]
- McKim KS, Green-Marroquin BL, Sekelsky JJ, Chin G, Steinberg C, Khodosh R, Hawley RS. 1998. Meiotic synapsis in the absence of recombination . Science 279 : 876–878. [ PubMed ] [ Google Scholar ]
- Meireles AM, Fisher KH, Colombié N, Wakefield JG, Ohkura H. 2009. Wac: A new Augmin subunit required for chromosome alignment but not for acentrosomal microtubule assembly in female meiosis . J Cell Biol 184 : 777–784. [ PMC free article ] [ PubMed ] [ Google Scholar ]
- Meyer RE, Kim S, Obeso D, Straight PD, Winey M, Dawson DS. 2013. Mps1 and Ipl1/Aurora B act sequentially to correctly orient chromosomes on the meiotic spindle of budding yeast . Science 339 : 1071–1074. [ PMC free article ] [ PubMed ] [ Google Scholar ]
- Morimoto A, Shibuya H, Zhu X, Kim J, Ishiguro K, Han M, Watanabe Y. 2012. A conserved KASH domain protein associates with telomeres, SUN1, and dynactin during mammalian meiosis . J Cell Biol 198 : 165–172. [ PMC free article ] [ PubMed ] [ Google Scholar ]
- Myers S, Bowden R, Tumian A, Bontrop RE, Freeman C, MacFie TS, McVean G, Donnelly P. 2010. Drive against hotspot motifs in primates implicates the PRDM9 gene in meiotic recombination . Science 327 : 876–879. [ PMC free article ] [ PubMed ] [ Google Scholar ]
- Nagaoka SI, Hodges CA, Albertini DF, Hunt PA. 2011. Oocyte-specific differences in cell-cycle control create an innate susceptibility to meiotic errors . Curr Biol 21 : 651–657. [ PMC free article ] [ PubMed ] [ Google Scholar ]
- Nakajo N, Yoshitome S, Iwashita J, Iida M, Uto K, Ueno S, Okamoto K, Sagata N. 2000. Absence of Wee1 ensures the meiotic cell cycle in Xenopus oocytes . Genes Dev 14 : 328–338. [ PMC free article ] [ PubMed ] [ Google Scholar ]
- Nasmyth K, Haering CH. 2009. Cohesin: Its roles and mechanisms . Annu Rev Genet 43 : 525–558. [ PubMed ] [ Google Scholar ]
- Nicolas A, Treco D, Schultes NP, Szostak JW. 1989. An initiation site for meiotic gene conversion in the yeast Saccharomyces cerevisiae . Nature 338 : 35–39. [ PubMed ] [ Google Scholar ]
- Niu H, Wan L, Busygina V, Kwon Y, Allen JA, Li X, Kunz RC, Kubota K, Wang B, Sung P, et al. 2009. Regulation of meiotic recombination via Mek1-mediated Rad54 phosphorylation . Mol Cell 36 : 393–404. [ PMC free article ] [ PubMed ] [ Google Scholar ]
- Ohi R, Sapra T, Howard J, Mitchison TJ. 2004. Differentiation of cytoplasmic and meiotic spindle assembly MCAK functions by Aurora B–dependent phosphorylation . Mol Biol Cell 15 : 2895–2906. [ PMC free article ] [ PubMed ] [ Google Scholar ]
- Oliver PL, Goodstadt L, Bayes JJ, Birtle Z, Roach KC, Phadnis N, Beatson SA, Lunter G, Malik HS, Pointing CP, 2010. Accelerated evolution of the Prdm9 speciation gene across diverse metazoan taxa . PLoS Genet 5 : e1000753. [ PMC free article ] [ PubMed ] [ Google Scholar ]
- Parfenov V, Potchukalina G, Dudina L, Kostyuchek D, Gruzova M. 1989. Human antral follicles: Oocyte nucleus and the karyosphere formation (electron microscopic and autoradiographic data) . Gamete Res 22 : 219–231. [ PubMed ] [ Google Scholar ]
- Parvanov ED, Petkov PM, Paigen K. 2010. Prdm9 controls activation of mammalian recombination hotspots . Science 327 : 835. [ PMC free article ] [ PubMed ] [ Google Scholar ]
- Petronczki M, Matos J, Mori S, Gregan J, Bogdanova A, Schwickart M, Mechtler K, Shirahige K, Zachariae W, Nasmyth K. 2006. Monopolar attachment of sister kinetochores at meiosis I requires casein kinase 1 . Cell 126 : 1049–1064. [ PubMed ] [ Google Scholar ]
- Pfender S, Kuznetsov V, Pleiser S, Kerkhoff E, Schuh M. 2011. Spire-type actin nucleators cooperate with Formin-2 to drive asymmetric oocyte division . Curr Biol 21 : 955–960. [ PMC free article ] [ PubMed ] [ Google Scholar ]
- Rabitsch KP, Petronczki M, Javerzat JP, Genier S, Chwalla B, Schleiffer A, Tanaka TU, Nasmyth K. 2003. Kinetochore recruitment of two nucleolar proteins is required for homolog segregation in meiosis I . Dev Cell 4 : 535–548. [ PubMed ] [ Google Scholar ]
- Radford SJ, Jang JK, McKim KS. 2012. The chromosomal passenger complex is required for meiotic acentrosomal spindle assembly and chromosome biorientation . Genetics 192 : 417–429. [ PMC free article ] [ PubMed ] [ Google Scholar ]
- Rao HB, Shinohara M, Shinohara A. 2011. Mps3 SUN domain is important for chromosome motion and juxtaposition of homologous chromosomes during meiosis . Genes Cells 16 : 1081–1096. [ PubMed ] [ Google Scholar ]
- Resnick TD, Dej KJ, Xiang Y, Hawley RS, Ahn C, Orr-Weaver TL. 2009. Mutations in the chromosomal passenger complex and the condensin complex differentially affect synaptonemal complex disassembly and metaphase I configuration in Drosophila female meiosis . Genetics 181 : 875–887. [ PMC free article ] [ PubMed ] [ Google Scholar ]
- Revenkova E, Herrmann K, Adelfalk C, Jessberger R. 2010. Oocyte cohesin expression restricted to predictyate stages provides full fertility and prevents aneuploidy . Curr Biol 20 : 1529–1533. [ PMC free article ] [ PubMed ] [ Google Scholar ]
- Ribbeck K, Groen AC, Santarella R, Bohnsack MT, Raemaekers T, Köcher T, Gentzel M, Görlich D, Wilm M, Carmeliet G, et al. 2006. NuSAP, a mitotic RanGTP target that stabilizes and cross-links microtubules . Mol Biol Cell 17 : 2646–2660. [ PMC free article ] [ PubMed ] [ Google Scholar ]
- Rieder CL, Salmon ED. 1994. Motile kinetochores and polar ejection forces dictate chromosome position on the vertebrate mitotic spindle . J Cell Biol 124 : 223–233. [ PMC free article ] [ PubMed ] [ Google Scholar ]
- Rieder CL, Davison EA, Jensen LC, Cassimeris L, Salmon ED. 1986. Oscillatory movements of monooriented chromosomes and their position relative to the spindle pole result from the ejection properties of the aster and half-spindle . J Cell Biol 103 : 581–591. [ PMC free article ] [ PubMed ] [ Google Scholar ]
- Riedel CG, Katis VL, Katou Y, Mori S, Itoh T, Helmhart W, Gálová M, Petronczki M, Gregan J, Cetin B, et al. 2006. Protein phosphatase 2A protects centromeric sister chromatid cohesion during meiosis I . Nature 441 : 53–61. [ PubMed ] [ Google Scholar ]
- Rivera T, Ghenoiu C, Rodríguez-Corsino M, Mochida S, Funabiki H, Losada A. 2012. Xenopus Shugoshin 2 regulates the spindle assembly pathway mediated by the chromosomal passenger complex . EMBO J 31 : 1467–1479. [ PMC free article ] [ PubMed ] [ Google Scholar ]
- Romanienko PJ, Camerini-Otero RD. 2000. The mouse Spo11 gene is required for meiotic chromosome synapsis . Mol Cell 6 : 975–987. [ PubMed ] [ Google Scholar ]
- Saito TT, Tougan T, Okuzaki D, Kasama T, Nojima H. 2005. Mcp6, a meiosis-specific coiled-coil protein of Schizosaccharomyces pombe , localizes to the spindle pole body and is required for horsetail movement and recombination . J Cell Sci 118 : 447–459. [ PubMed ] [ Google Scholar ]
- Sakuno T, Tada K, Watanabe Y. 2009. Kinetochore geometry defined by cohesion within the centromere . Nature 458 : 852–858. [ PubMed ] [ Google Scholar ]
- Sampath SC, Ohi R, Leismann O, Salic A, Pozniakovski A, Funabiki H. 2004. The chromosomal passenger complex is required for chromatin-induced microtubule stabilization and spindle assembly . Cell 118 : 187–202. [ PubMed ] [ Google Scholar ]
- Sato A, Isaac B, Phillips CM, Rillo R, Carlton PM, Wynne DJ, Kasad RA, Dernburg AF. 2009. Cytoskeletal forces span the nuclear envelope to coordinate meiotic chromosome pairing and synapsis . Cell 139 : 907–919. [ PMC free article ] [ PubMed ] [ Google Scholar ]
- Scherthan H, Weich S, Schwegler H, Heyting C, Härle M, Cremer T. 1996. Centromere and telomere movements during early meiotic prophase of mouse and man are associated with the onset of chromosome pairing . J Cell Biol 134 : 1109–1125. [ PMC free article ] [ PubMed ] [ Google Scholar ]
- Schuh M, Ellenberg J. 2008. A new model for asymmetric spindle positioning in mouse oocytes . Curr Biol 18 : 1986–1992. [ PubMed ] [ Google Scholar ]
- Shao H, Li R, Ma C, Chen E, Liu XJ. 2013. Xenopus oocyte meiosis lacks spindle assembly checkpoint control . J Cell Biol 201 : 191–200. [ PMC free article ] [ PubMed ] [ Google Scholar ]
- Silljé HH, Nagel S, Körner R, Nigg EA. 2006. HURP is a Ran-importin β-regulated protein that stabilizes kinetochore microtubules in the vicinity of chromosomes . Curr Biol 16 : 731–742. [ PubMed ] [ Google Scholar ]
- Sollier J, Lin W, Soustelle C, Suhre K, Nicolas A, Géli V, de La Roche Saint-André C. 2004. Set1 is required for meiotic S-phase onset, double-strand break formation and middle gene expression . EMBO J 23 : 1957–1967. [ PMC free article ] [ PubMed ] [ Google Scholar ]
- Tachibana-Konwalski K, Godwin J, van der Weyden L, Champion L, Kudo NR, Adams DJ, Nasmyth K. 2010. Rec8-containing cohesin maintains bivalents without turnover during the growing phase of mouse oocytes . Genes Dev 24 : 2505–2516. [ PMC free article ] [ PubMed ] [ Google Scholar ]
- Tanaka S, Araki H. 2010. Regulation of the initiation step of DNA replication by cyclin-dependent kinases . Chromosoma 119 : 565–574. [ PubMed ] [ Google Scholar ]
- Tanaka K, Kohda T, Yamashita A, Nonaka N, Yamamoto M. 2005. Hrs1p/Mcp6p on the meiotic SPB organizes astral microtubule arrays for oscillatory nuclear movement . Curr Biol 15 : 1479–1486. [ PubMed ] [ Google Scholar ]
- Tanno Y, Kitajima TS, Honda T, Ando Y, Ishiguro K, Watanabe Y. 2010. Phosphorylation of mammalian Sgo2 by Aurora B recruits PP2A and MCAK to centromeres . Genes Dev 24 : 2169–2179. [ PMC free article ] [ PubMed ] [ Google Scholar ]
- Theurkauf WE, Hawley RS. 1992. Meiotic spindle assembly in Drosophila females: Behavior of nonexchange chromosomes and the effects of mutations in the nod kinesin-like protein . J Cell Biol 116 : 1167–1180. [ PMC free article ] [ PubMed ] [ Google Scholar ]
- Tóth A, Rabitsch KP, Gálová M, Schleiffer A, Buonomo SB, Nasmyth K. 2000. Functional genomics identifies monopolin: A kinetochore protein required for segregation of homologs during meiosis I . Cell 103 : 1155–1168. [ PubMed ] [ Google Scholar ]
- Tseng BS, Tan L, Kapoor TM, Funabiki H. 2010. Dual detection of chromosomes and microtubules by the chromosomal passenger complex drives spindle assembly . Dev Cell 18 : 903–912. [ PMC free article ] [ PubMed ] [ Google Scholar ]
- Uhlmann F, Nasmyth K. 1998. Cohesion between sister chromatids must be established during DNA replication . Curr Biol 8 : 1095–1101. [ PubMed ] [ Google Scholar ]
- Wainman A, Buster DW, Duncan T, Metz J, Ma A, Sharp D, Wakefield JG. 2009. A new Augmin subunit, Msd1, demonstrates the importance of mitotic spindle-templated microtubule nucleation in the absence of functioning centrosomes . Genes Dev 23 : 1876–1881. [ PMC free article ] [ PubMed ] [ Google Scholar ]
- Watanabe Y, Nurse P. 1999. Cohesin Rec8 is required for reductional chromosome segregation at meiosis . Nature 400 : 461–464. [ PubMed ] [ Google Scholar ]
- Watanabe Y, Yokobayashi S, Yamamoto M, Nurse P. 2001. Pre-meiotic S phase is linked to reductional chromosome segregation and recombination . Nature 409 : 359–363. [ PubMed ] [ Google Scholar ]
- Wiese C, Wilde A, Moore MS, Adam SA, Merdes A, Zheng Y. 2001. Role of importin-β in coupling Ran to downstream targets in microtubule assembly . Science 291 : 653–656. [ PubMed ] [ Google Scholar ]
- Wignall SM, Villeneuve AM. 2009. Lateral microtubule bundles promote chromosome alignment during acentrosomal oocyte meiosis . Nat Cell Biol 11 : 839–844. [ PMC free article ] [ PubMed ] [ Google Scholar ]
- Wynne DJ, Rog O, Carlton PM, Dernburg AF. 2012. Dynein-dependent processive chromosome motions promote homologous pairing in C. elegans meiosis . J Cell Biol 196 : 47–64. [ PMC free article ] [ PubMed ] [ Google Scholar ]
- Yamamoto A, West RR, McIntosh JR, Hiraoka Y. 1999. A cytoplasmic dynein heavy chain is required for oscillatory nuclear movement of meiotic prophase and efficient meiotic recombination in fission yeast . J Cell Biol 145 : 1233–1249. [ PMC free article ] [ PubMed ] [ Google Scholar ]
- Yao Y, Dai W. 2012. Shugoshins function as a guardian for chromosomal stability in nuclear division . Cell Cycle 11 : 2631–2642. [ PMC free article ] [ PubMed ] [ Google Scholar ]
- Yi K, Unruh JR, Deng M, Slaughter BD, Rubinstein B, Li R. 2011. Dynamic maintenance of asymmetric meiotic spindle position through Arp2/3-complex-driven cytoplasmic streaming in mouse oocytes . Nat Cell Biol 13 : 1252–1258. [ PMC free article ] [ PubMed ] [ Google Scholar ]
- Yokobayashi S, Watanabe Y. 2005. The kinetochore protein Moa1 enables cohesion-mediated monopolar attachment at meiosis I . Cell 123 : 803–817. [ PubMed ] [ Google Scholar ]
- Zuccotti M, Giorgi Rossi P, Martinez A, Garagna S, Forabosco A, Redi CA. 1998. Meiotic and developmental competence of mouse antral oocytes . Biol Reprod 58 : 700–704. [ PubMed ] [ Google Scholar ]
- Zuccotti M, Ponce RH, Boiani M, Guizzardi S, Govoni P, Scandroglio R, Garagna S, Redi CA. 2002. The analysis of chromatin organisation allows selection of mouse antral oocytes competent for development to blastocyst . Zygote 10 : 73–78. [ PubMed ] [ Google Scholar ]
Thank you for visiting nature.com. You are using a browser version with limited support for CSS. To obtain the best experience, we recommend you use a more up to date browser (or turn off compatibility mode in Internet Explorer). In the meantime, to ensure continued support, we are displaying the site without styles and JavaScript.
- View all journals
- Explore content
- About the journal
- Publish with us
- Sign up for alerts
- RESEARCH BRIEFINGS
- 02 August 2024
Chromosome components central to cell division evolve rapidly
This is a summary of: Logsdon, G. A. et al . The variation and evolution of complete human centromeres. Nature 629 , 136–145 (2024) .
Access options
Access Nature and 54 other Nature Portfolio journals
Get Nature+, our best-value online-access subscription
24,99 € / 30 days
cancel any time
Subscribe to this journal
Receive 51 print issues and online access
185,98 € per year
only 3,65 € per issue
Rent or buy this article
Prices vary by article type
Prices may be subject to local taxes which are calculated during checkout
doi: https://doi.org/10.1038/d41586-024-02523-1
‘Expert opinion’ and the figure are published under a CC BY 4.0 licence.
Nurk, S. et al. Science 376 , 44–53 (2022).
Article PubMed Google Scholar
Altemose, N. et al. Science 376 , eabl4178 (2022).
Logsdon, G. A. et al. Nature 593 , 101–107 (2021).
Henikoff, S., Ahmad, K. & Malik, H. S. Science 293 , 1098–1102 (2001).
Liao, W.-W. et al. Nature 617 , 312–324 (2023).
Download references
Reprints and permissions
Related Articles
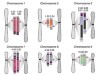
- DNA sequencing
- Cell biology
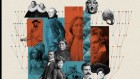
From Vikings to Beethoven: what your DNA says about your ancient relatives
News Feature 07 AUG 24
The pandemic agreement: an African perspective
Correspondence 16 JUL 24
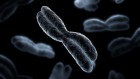
First fossil chromosomes discovered in freeze-dried mammoth skin
News 11 JUL 24
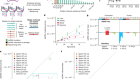
DNA mismatch and damage patterns revealed by single-molecule sequencing
Article 12 JUN 24
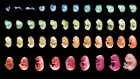
One year, three researchers, millions of cells: how a small team created the largest mouse-embryo atlas so far
Technology Feature 20 MAR 24
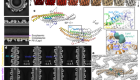
Cryo-EM architecture of a near-native stretch-sensitive membrane microdomain
Article 24 JUL 24
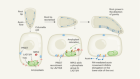
Things fall into place: how plants sense and respond to gravity
News & Views 17 JUL 24
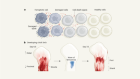
Waves of ferroptotic cell death sculpt embryonic tissue
News & Views 10 JUL 24
Faculty Positions in Infection Biology, Microbiomics, and Infectious Disease Modelling
The Bioscience Program in the Biological and Environmental Science and Engineering (BESE) Division
Saudi Arabia (SA)
King Abdullah University of Science and Technology (KAUST
Gathering the Talents and Writing Our Glorious Chapter
Wuxi Medical College of Jiangnan University (Affiliated Hospital) invites you to join us!
Wuxi, Jiangsu (CN)
Wuxi Medical College of Jiangnan University (Affiliated Hospital)
AI for Biomolecular Sciences Researcher
Xiaoliang Sunney XIE’s Group is recruiting researchers specializing in AI for Biomolecular Science.
Beijing (CN)
Changping Laboratory
Post-Doctoral Fellowship in Regenerative Biology and Medicine (Lab of. Dr. Yuval Rinkevich)
Discovery of cellular and molecular mechanisms of tissue repair and regeneration.
Beijing, China
Institute for Regenerative Biology and Medicine, Chinese Institutes for Medical Research (CIMR)
Open Faculty Positions at the State Key Laboratory of Brain Cognition & Brain-inspired Intelligence
The laboratory focuses on understanding the mechanisms of brain intelligence and developing the theory and techniques of brain-inspired intelligence.
Shanghai, China
CAS Center for Excellence in Brain Science and Intelligence Technology (CEBSIT)
Sign up for the Nature Briefing newsletter — what matters in science, free to your inbox daily.
Quick links
- Explore articles by subject
- Guide to authors
- Editorial policies
- Open access
- Published: 03 August 2024
The interplay of transition metals in ferroptosis and pyroptosis
- Frantisek Vana 2 ,
- Zoltan Szabo 2 , 4 ,
- Michal Masarik 1 , 2 , 3 &
- Monika Kratochvilova 1
Cell Division volume 19 , Article number: 24 ( 2024 ) Cite this article
37 Accesses
Metrics details
Cell death is one of the most important mechanisms of maintaining homeostasis in our body. Ferroptosis and pyroptosis are forms of necrosis-like cell death. These cell death modalities play key roles in the pathophysiology of cancer, cardiovascular, neurological diseases, and other pathologies. Transition metals are abundant group of elements in all living organisms. This paper presents a summary of ferroptosis and pyroptosis pathways and their connection to significant transition metals, namely zinc (Zn), copper (Cu), molybdenum (Mo), lead (Pb), cobalt (Co), iron (Fe), cadmium (Cd), nickel (Ni), mercury (Hg), uranium (U), platinum (Pt), and one crucial element, selenium (Se). Authors aim to summarize the up-to-date knowledge of this topic.
In this review, there are categorized and highlighted the most common patterns in the alterations of ferroptosis and pyroptosis by transition metals. Special attention is given to zinc since collected data support its dual nature of action in both ferroptosis and pyroptosis. All findings are presented together with a brief description of major biochemical pathways involving mentioned metals and are visualized in attached comprehensive figures.
This work concludes that the majority of disruptions in the studied metals’ homeostasis impacts cell fate, influencing both death and survival of cells in the complex system of altered pathways. Therefore, this summary opens up the space for further research.
Graphical abstract

Introduction
In this review, we aim to describe the role of transition metals in ferroptosis and pyroptosis. Ferroptosis and pyroptosis represent two distinct modes of regulated necrotic cell death, both of which have gained recognition in recent years. Given that cellular death plays a pivotal role in maintaining the homeostasis in our body, their importance is acknowledged across various research domains. These include embryology, evolution and developmental biology, various disease pathologies or novel therapy and drug discovery. Like all cellular processes, programmed cell death can be affected by external or internal factors.
One influential factor is the presence of transition metals, typically found in small quantities, making even minor fluctuations. They could be a critical determinant in the execution of ferroptotic and pyroptotic cell deaths. Consequently, these changes can have either beneficial or detrimental effects on the organism, depending on the specific circumstances.
We selected papers for this review based on their relevance to the topic, and therefore these studies have a great variability of experimental models (animals, cell lines). Because not all studies were performed on the same scientific model, some comparisons are not possible. Moreover, pyroptosis is type of cell death prominent only for cells of the immune system. However, this paper focuses on the resume of current state of knowledge.
Understanding the intricate relationships between cell death pathways and transition metals holds the potential to enhance our comprehension of health maintenance, disease prevention, and treatment. For example, modulating transition metal levels or targeting metal-related pathways can potentially be used to regulate these forms of cell death.
Today, the ever-growing importance of TMs (transition metals) in industrial usage means the possible exposure to these exotic materials in various forms. This opens another field for further research of the interactions yet unknown.
- Ferroptosis
Discovery of ferroptosis
The initial research that caught attention of biomedicine scientists’ dates to 2003. In this study the ferroptosis-inducing substance erastin was recognised as an effective drug to kill tumourtransformed fibroblasts with the expression of oncoprotein K-Ras (Kirsten rat sarcoma virus) [ 1 ]. However, this early on, ferroptosis itself was not yet discovered. Ferroptosis is a recently defined necrosis-like cell death, first described by Dixon et al. in 2012 [ 2 ]. In their study, cell death was induced by erastin and inhibited by iron chelators, which underlines the crucial role of iron in this cell death mechanism [ 3 , 4 ]. The cystine/glutamate transport system (Xc system) was further identified as a target of erastin [ 3 , 4 ]. This system transports glutamate out of the cell in exchange for cystine, which is then used by the cell to synthesize glutathione (GSH, γ-Glutamylcysteinylglycine, non-protein thiol). Erastin treatment leads to the exhaustion of cellular GSH deposit and inhibits glutathione peroxidase 4 (GPX4). GPX4 prevents the peroxidation of lipids and usually downgrades the autoactivation of ferroptosis in the cell [ 3 , 4 ]. The most important ferroptosis pathways are shown in the Fig. 1 .
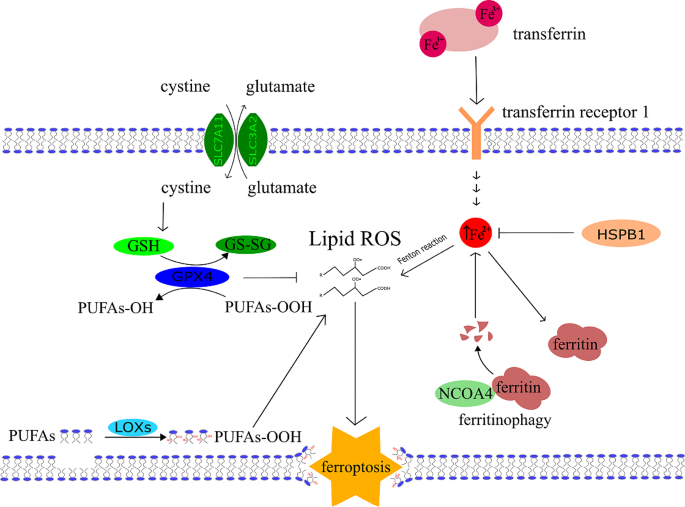
General mechanism of ferroptosis. Three main axes are described in this scheme. The first is an inhibitory axis centred around GPX4, which can convert lipid hydroperoxide into lipid alcohols. GPX4 needs glutathione (GSH) as a cofactor and its main component, cysteine, is delivered through the Xc-cystine-glutamate antiport system, which consists of two units SLC7A11 (Solute Carrier Family 7 Member 11) and SLC32A2 (Solute Carrier Family 32 Member 2). The second axis represents iron metabolism and the capability of iron to undergo the Fenton reaction, which is the main source of ROS reacting with lipids. Fe 2+ reacts with hydroperoxide and generates the most potent oxygen radical, the hydroxyl radical. The third axis consists of lipoxygenases, and their substrates – PUFAs. Phosphorylated Heat shock protein 1 (HSPB1) acts as a negative regulator of ferroptosis by reducing cellular iron uptake and lipid ROS production [ 281 ]. Another factor elevating the Fe 2+ pool is ferritinophagy, which is mediated by a selective cargo receptor Nuclear receptor coactivator 4 (NCOA4). The third axis is driven by lipoxygenases (LOXs) which allow enzymatic oxidation of PUFAs to form. PUFAs-OOH adding to the lipid ROS pool. All three mentioned axes increase the amount of lipid ROS in the membrane which then leads to its rupture and ferroptosis occurs
Execution of ferroptosis
Oxidative stress induces ferroptosis by the depletion of cellular antioxidative potential [ 5 ]. Reactive oxygen species (ROS) represent the driving force of oxidative stress [ 6 ]. The main source of ROS, the Fenton reaction, will be discussed further. Polyunsaturated fatty acids (PUFAs), found mainly in plasmatic membranes, undergo peroxidation due to oxidative stress [ 7 ]. This process leads to the rupture of the plasmatic membrane followed by osmolysis, which completes the ferroptotic death of cells [ 7 ].
Lipoxygenases and ferroptosis
Lipoxygenases (LOX) are enzymes that transform cholesterol and PUFAs (mainly arachidonic acid) found in cytoplasmatic membranes into hydroperoxide derivates and therefore contribute to lipid peroxidation [ 8 ]. Together with free radical-driven, iron-dependent Fenton reaction, the LOX activity creates the pool of lipid radicals, the hallmark of ferroptosis [ 9 , 10 , 11 ]. Lipoxygenases will also be further discussed in relation to iron and ferroptosis.
Mitochondria and ferroptosis
Typical morphological signs of ferroptosis are small mitochondria with condensed membranes and with the disappearance of the mitochondria cristae or with the rupture of the membranes [ 12 ]. Shrunken or ruptured mitochondria are one of the key morphological signs of occurring ferroptosis and therefore mitochondria are supposed to be related to ferroptosis [ 13 ]. The exact relationship is unclear since the depletion of mitochondria does not seem to have a clear effect on ferroptosis. In one study, the cells depleted of mitochondria could still undergo ferroptosis [ 14 ], while in another study authors described significantly attenuated ferroptosis [ 3 ]. Mitochondria have their own GPX4, but its inhibition does not typically induce strong lipid peroxidation in mitochondria and the ferroptosis driving lipid peroxidation appears mainly in the cytoplasm [ 15 ]. Mitochondria can contribute to ferroptosis in other ways, including ROS generation as a byproduct of oxidative phosphorylation. Specifically strong ROS generation is caused by electron leakage from respiratory chain complexes I and III. Such action produces hydrogen peroxide (H 2 O 2 ) by the activity of superoxide dismutase (SOD)mediated dismutation [ 16 ]. H 2 O 2 can then react with Fe 2+ and produce hydroxyl radical which then reacts with the bis-allylic hydrogen in the structure of PUFAs and PUFA radicals are produced and can propagate [ 16 ]. Nevertheless, the detailed role of mitochondria in ferroptotic cell death is beyond the scope of this paper, see other authors review [ 12 , 17 ].
Distinction between oxytosis and ferroptosis
In the absence of glutamine or during the inhibition of glutaminolysis the erastin-induced blockade of cystine import can lead to cell death. α-ketoglutarate, a product of glutaminolysis, can substitute the glutamine during the induction of ferroptosis [ 18 ]. High levels of glutamine in the extracellular fluid can induce ferroptosis via the inhibition of cystine uptake by the Xc- system [ 19 ]. This phenomenon was described in the cells of the central nervous system and was proven to be different from apoptosis and was given a name oxytosis [ 20 ]. Oxytosis has some similar features to ferroptosis, such as cysteine and GSH deprivation or ROS formation and lipid peroxidation, but differs in the terminal phase, which may be executed with the signs of apoptosis and is more dependent on the calcium than on iron [ 21 ].
The importance of ferroptosis in health and diseases
The physiological role of ferroptosis is not clear, but some studies suggest its importance during the development of the immune system, since the correct embryonal development of the immune system in humans depends on the adequate intake of PUFAs [ 22 ]. This was further supported by the study where T-lymphocytes without functional GPX4 were not capable of clonal expansion and did not protect against choriomeningitis virus or parasitical Leishmanias infection [ 23 ].
While ferroptosis has been linked to several diseases, it has been mainly studied in the context of cardiomyopathy [ 24 ], and in the context of neurological conditions, such as stroke, ischemia reperfusion injury or Parkinson´s, Huntington´s [ 25 ] and Alzheimer´s disease [ 4 , 26 , 27 ]. The occurrence in cardiomyopathy can be supported by the recent studies indicating that enhancing the glutathione system (the biochemical antioxidative system centred around glutathione [ 28 ]) and inhibiting ferroptosis, a cell death process intricately connected to the GSH system, holds a significant potential as a therapeutic approach for numerous cardiac diseases [ 29 ].
In Alzheimer´s disease, the role of ferroptosis is multimodal, but mainly connected to altered lipid metabolism and iron homeostasis. In experimental study on murine model, Bao and his team injected Amyloid Aβ into the brains of mice [ 30 ]. They noticed elevated levels of iron and ferritin in the hippocampus, along with decreased levels of GPX4. This finding shows one of the possible ways of ferroptosis involvement in the pathogenesis of Alzheimer´s disease.
Following cerebral ischemia, the blood-brain barrier (BBB) loses its tight junctional integrity, leading to disruption. This permits the entry of Fe 3+ from the bloodstream into the brain parenchyma facilitated by transferrin (TF) and transferrin receptor (TFR) [ 31 ]. Fe 3+ is subsequently reduced to Fe 2+ and via the Fenton reaction generates ROS, promoting ferroptosis.
When reperfusion occurs after an ischemic period, excitatory amino acids represented by glutamate accumulate in the synaptic cristae. This leads to a decline in glutamate intake and an increase in the extracellular glutamate release, effectively inhibiting the Xc − system [ 32 ]. This all then enhances the ferroptosis during stroke ischemia and reperfusion in the brain [ 33 ].
Ferroptosis has been recognised as an important tumour-suppressing mechanism as well [ 34 ], and the evidence of its significance in oncological treatment is expanding each year [ 17 ]. All these information only highlight the importance of ferroptosis in health and disease. Detailed description would be topic large enough for another comprehensive review. However, a review on mechanisms of ferroptosis in related diseases has already been written by Feng, Tang et al. In this paper, authors discuss ferroptosis in great dept in each organ system [ 35 ].
Discovery of pyroptosis
Pyroptosis is a modality of proinflammatory, regulated, necrotic-like cell death. Its cascade is centred around human Caspase-1, Caspase-4, and Caspase-5 or murine Caspase-11 [ 36 ]. They all belong to the family of proinflammatory Caspases and are essential for adequate reaction to pathogens [ 37 ]. The first observation of pyroptosis was during an experiment in which macrophages were infected with Salmonella enterica serovar Typhimurium (S. Typhimurium) or Shigella flexneri [ 38 , 39 ]. During pyroptosis, proinflammatory Caspases (except for Caspase-12) activate on behalf of the inflammasome protein complex (see further) [ 40 , 41 , 42 ].

Tissue and cell specificity of pyroptosis
Not all cell types can undergo pyroptosis. This type of cell death is prominent for cells of the immune system, most notably macrophages, since canonical pyroptosis depends on the presence of inflammasomes [ 43 ]. Apart from immune system cells, pyroptosis has been documented in endothelial, neuronal cells, also in jejunal a renal epithelial cells and in various cancer cells (melanoma, osteosarcoma, colon carcinoma, lung cancer, triple negative breast cancer, cervical cancer, nasopharyngeal and oesophageal cancer cells) [ 11 , 44 , 45 , 46 , 47 , 48 , 49 , 50 , 51 , 52 , 53 , 54 , 55 , 56 , 57 , 58 , 59 ].
Pyroptosis and imunity and inflammation
Pyroptosis has an important role in the activation of inflammation due to the release of the proinflammatory interleukins IL-1β and IL-18 [ 60 ]. This is accompanied by the release of DAMPs (damage-associated molecular patterns) [ 61 ] which enhance the stimulation of proinflammatory action, including the recruitment and activation of neutrophils, macrophages and other immune cells [ 62 ]. If the exposure to the proinflammatory factors is chronic, it can lead to the development of autoimmune diseases [ 63 ]. Some bacteria can replicate in macrophages in which pyroptosis is subsequently triggered. Pyroptosis leads to the termination of bacterial replication cycle and to the formation of PITs (pore-induced intracellular traps), that cage the bacteria in the fragments of the dead macrophage. Ligands of the PITs are recognised by neutrophils, triggering them to efferocytosis and to eliminate the pathogen [ 64 ].
Canonical and noncanonical pathways of pyroptosis
Pyroptosis can be activated via mechanisms of canonical and noncanonical signalling pathway. The canonical pathway starts when DAMPs or PAMPs (pathogen associated molecular patterns) stimulate the formation of inflammasomes, which lead to the forming of pyroptosome and Caspase-1 activation [ 65 ]. The noncanonical pathway is induced by endotoxins produced by gramnegative bacteria (for example lipopolysaccharides (LPS) as portrayed in Fig. 2 ) [ 66 ]. Those endotoxins bind directly to the human proCaspase-4 [ 67 ]. In the murine model, PAMPs activate the proCaspase-11, homologous to the human proCaspase-4 and − 5 [ 66 ]. Both canonical and noncanonical pathways are visualised in Fig. 2 . Based on evidence, it appears that the activation of specific proapoptotic Caspase cascades may be also required during pyroptosis [ 68 ]. This activation occurs due to BAX (bcl-2-like protein 4) activation, which leads to the permeabilization of the mitochondrial outer membrane [ 68 ]. Caspases activated during both canonical and noncanonical pathways cleave gasdermin D (GSDMD) [ 69 , 70 , 71 , 72 ]. The product of the cleavage, the N-terminal fragment of GSDMD, then oligomerizes in membranes to form pores causing necrotic-like cell death [ 73 , 74 , 75 , 76 ].
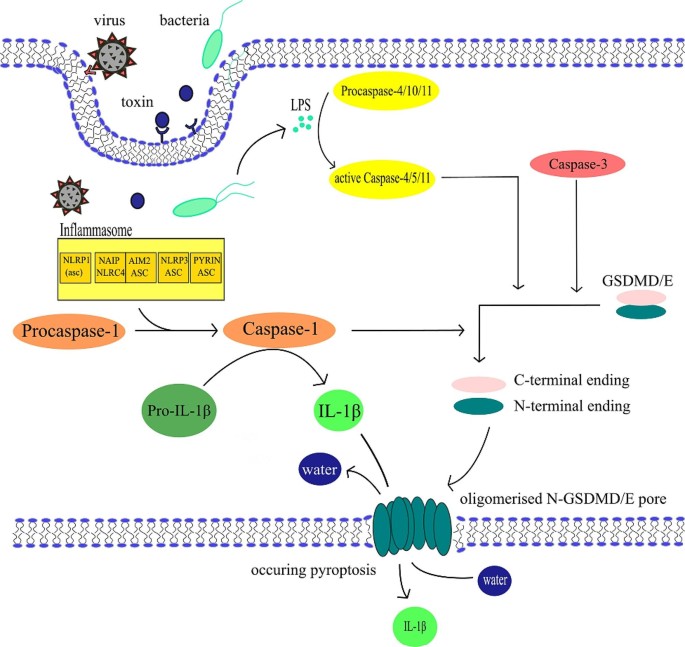
General pyroptosis mechanism – canonical and noncanonical pathway. Canonical pathway is centred around the inflammasome in this figure. Stimulation of this non-membranous organelle leads to the activation of Caspase-1, which then turns pro-IL-1β into IL-1β and cleaves gasdermins GSDME or GSDMD into N- and C-terminal ending. In membranes, the N‑terminal protein residues of GSDMs polymerise to form pores, causing water and electrolyte leakage and pyroptosis. IL-1β leaves the cell through these pores. Noncanonical pathway starts with LPS activating proCaspases − 4/-5/-10, which in their active forms are capable of cleaving gasdermins as well. During the activation of Caspase-3 the mechanism of cell death is decided by the expression level of GSDME. High levels of GSDME switch apoptosis to pyroptosis with oligomerised N‑GSDME pore formation [ 83 ]
Gasdermins in pyroptosis
There are more gasdermins than gasdermin-D (GSDMD) and gasdermin-E (GSDME), that can participate in the activation of pyroptosis. Namely, gasdermin A (GSDMA) and gasdermin B (GSDMB) [ 77 , 78 , 79 ]. The cleavage of GSDME can be, in some situations, mediated by Caspase-3. Caspase-3 activators include certain chemotherapeutics, TNF-α (tumour necrosis factor) and Caspase-8, that usually would induce apoptosis. However, the Caspase-3/GSDME pathway can switch apoptosis to pyroptosis when the levels of GSDME are increased [ 80 , 81 ]. This deviation is responsible for many side effects of certain chemotherapeutics [ 82 ], probably because of increased activation of pyroptosis in healthy cells. GSDME is typically expressed in healthy tissues, but tumour cells often lack GDSME [ 83 ]. On the other hand, in the absence of gasdermins, Caspase-1 can activate Caspase-3, Caspase-8 and Caspase-7 to start apoptosis, that serves as a reserve cell death mechanism in the case of pyroptosis insufficiency [ 84 ].
Inflammasomes
Inflammasomes are non-membranous organelles and are distinguished by the presence of receptors (inflammasome assembling PRRs – pattern recognition receptors) [ 80 ]. Such inflammasome assembling PRRs are expressed in many cell types, mainly in immune cells (macrophages, dendritic cells, neutrophils and epithelial cells) [ 85 ]. Innate immunity relies on inflammasomes, which are composed of intracellular sensors coupled with Caspase and interleukin activating systems [ 86 ]. Inflammasomes trigger pyroptosis and activate inflammatory cascades. NLRP3 (nucleotide-binding oligomerization domain-like receptor protein), NLRP6, NLRC4, and AIM2 (absent in melanoma) are prominent inflammasome members [ 86 ].
Two main types of inflammasomes were observed. Inflammasomes containing NOD-like receptors (NLR) and inflammasomes containing PYHIN (Pyrin and HIN domain) family members [ 87 , 88 ]. NLR receptors are receptors containing nucleotide-binding oligomerizing domains [ 65 ]. This group of inflammasomes consists of NLRP3 (NLR family pyrin domain containing 3) based inflammasomes, inflammasomes based on NLRP1, and inflammasomes based on NLRC4 (NLR family CARD domaincontaining protein 4) [ 65 ]. It was observed, that other NLR proteins such as NLRP6 and NLRP7 can participate on the same process as well [ 65 ]. To the other (PYHIN) group of inflammasomes belong inflammasomes based on AIM2 (absent in melanoma 2) and IFI16 (interferoninducible protein 16) [ 65 , 89 ].
ROS are essential inflammasome activating signals, activating inflammasomes through MAPK (mitogen activated protein kinase) and ERK1/2 (extracellular signal regulated kinase) [ 86 ]. Many of the transition metals have some relation to redox homeostasis and can be connected to pyroptosis with the repeating pattern of ROS activated pyroptosis [ 86 ]. Inflammasome dysregulation contributes to various diseases, including viral infections, silicosis, gout, and diabetes [ 90 ]. SIRT-1 (sirtuin-1) antioxidants can reduce inflammasome activation [ 90 ]. Inflammasomes and related pathways are potential therapeutic targets for various pathological conditions [ 86 ].
Pyroptosome
Pyroptosome is a large subcellular structure, which can be visualized via fluorescent staining of ASC (apoptosis associated speck-like protein containing a CARD, caspase activation and recruitment domain) [ 91 ]. It is important to note that pyroptosome is not necessary for the execution of pyroptosis, which can occur by NLRC4 dependent Caspase-1 activation [ 92 ]. Pyronecrosis, a pyroptosis subtype that can be triggered by Shigella gonorrhoeae or Neisseria gonorrhoeae is NLRP3 dependent and does not require activation by Caspase-1 [ 93 , 94 ].
The importance of pyroptosis in health and diseases
As mentioned above, pyroptosis holds great importance in inflammation and immunity. Logically, any malfunction in the form of hyper- or hypo- activation can lead to various health problems. Especially among autoimmune diseases, we can distinguish NLR-3 related systemic autoinflammatory diseases (SAIDs).
The abnormal activation of inflammasomes is a significant factor driving SAIDs and hence pyroptosis may also contribute to SAIDs. The evidence indicates that the absence of GSDMD does not affect the activation of pro-IL-1β into IL-1β but rather hampers the release of IL-1β. This suggests that in SAIDs patients, the inflammatory response originates from excessive cytokine release via pores during pyroptosis [ 95 ]. Nevertheless, IL-1β can be released by other mechanisms than pyroptosis, and thus the connection between pyroptosis, IL-1β, and SAIDs does not need to be direct.
In some cases, the evidence for pyroptosis impacting the SAIDs is solid and supported by genetically distinct groups of SAIDs with relation to NLRP-3 and pyrin [ 96 ]. The list of NLRP-3 related SAIDs includes cryopyrin-associated periodic syndrome (CAPS), gout, and Crohn’s disease [ 97 ]. List of Pyrin associated SAIDs contains familial mediterranean fever (FMF), pyrin-associated auto-inflammation with neutrophilic dermatosis (PAAND) and livedoid ulcerative dermatitis [ 98 , 99 , 100 ].
Pyroptosis appears to play an important role in Covid-19 pneumonia, arthritis and other inflammatory diseases as well [ 101 , 102 , 103 , 104 ]. For other details on this topic, we recommend a review published in Nature [ 105 ].
Pyroptosis has importance in cancer biology as well, since immune system-mediated pro-cancer and anti-cancer mechanisms play a significant role in carcinogenesis. Pyroptosis can have a dual effect on tumours [ 106 ], it can suppress their occurrence and expansion, or on the other hand help with the development of a suitable microenvironment for tumour’s cell growth. The extended exposure to an inflammatory environment raises the risk of cancer development according to cell and tissue level theory of carcinogenesis. In particular, cytokines released during pyroptosis, such as IL-1β and IL-18, can promote tumour infiltration, thereby increasing the chances of tumorigenesis and metastasis [ 107 , 108 ]. On the other hand, the anti-cancer activity of pyroptosis has not been fully understood yet and is still subject of research [ 106 ]. On the topic of pyroptosis’ dual role in cancer we recommend a great review published in Cellular & Molecular immunology journal [ 106 ].
The importance of transition metals
Signalling is on any scale dependent on the location [ 109 ]. The medicinal compounds containing metals undergo metabolism to produce simple metal ions, which then interact with cellular components to generate novel compounds. These newly formed compounds may exhibit biological activity, thereby prolonging the therapeutic effects of the initial drug introduced into the system [ 110 ]. Thanks to this quality, they can contribute to signalling through coordinated spatial and temporal changes in their local concentrations and ligation statuses. These involve movements, redox changes or catalysis and ligand alterations on different levels [ 111 ].
Altogether, transition metals have various roles in cells, which are the following:
cofactors and structural part of enzymes [ 112 ],
maintenance of protein conformations [ 113 ],
mediation enzyme activation/inhibition [ 114 ],
direct participation in redox homeostasis,
second messengers [ 115 ].
The state of the cellular metal pool is important [ 111 ]. But the identification of TMs’ targets of signalling is even more crucial [ 116 ]. However, both target prediction and identification are difficult, since the diversity and complexity of the metal sites is high [ 117 ]. To get the broader understanding of transition metal signalling see a short review: Searching for harmony in transition-metal signalling [ 111 ].
TMs have proven relation to autophagy, a metabolic program closely related to pyroptosis and ferroptosis. In various studies, autophagy incidence grew after the cells were treated with heavy metals mostly found in the group of TMs [ 118 ]. And thus, this paper provides short biochemical highlights of TMs, concerning the facts connecting TMs to ferroptosis and pyroptosis.
Iron is an essential element for life [ 119 ], occurring mainly in its bound forms. Heme complexes, ironcontaining tetrapyrroles, are the most abundant form of iron in the human body [ 120 ]. Since heme is toxic in excess, it is bound to proteins called heme proteins, which also form heme enzymes. These facilitate gas transportation, oxidative and xenobiotic metabolism [ 120 ]. Iron-sulphur clusters (Fe-S) function as essential parts of the respiratory chain (in mitochondria) and photosynthesis (in plastids) [ 121 ]. Iron bound to proteins in non-heme form represents the main transport and storage form of iron, which will be discussed further. Iron chelators are iron-binding compounds, which affect the availability of iron. They may decrease iron uptake when present in food (for example, phytate and polyphenols) [ 122 ], and are used to treat iron-overload syndromes [ 123 ]. Recently, iron chelators are emerging as a treatment of diseases associated with oxidative stress, including atherosclerosis, cardiovascular and neurodegenerative diseases, and cancer [ 124 ]. Free state iron occurs in Fe 2+ and Fe 3+ forms. Balance between these two oxidative states plays a key role in maintaining of the redox balance in cells, which requires strict free iron pool regulation [ 125 ].
The uptake of dietary iron happens in the small intestine [ 126 ]. Heme bound iron entry is still not well understood [ 120 ]. Two potential mechanisms have been suggested, either a receptor-mediated endocytosis and membrane transport via a transporter [ 127 ], or a transporter mediated entry via HRG1 (heme responsive gene). This transporter was found on erythrophagosomal membranes of macrophages during iron scavenging from red blood cells [ 128 ]. However, enterocytes of the human small intestine also express HRG1, where it could serve as a heme importer from endocytic compartments [ 129 ]. Heme oxygenase (HO) then splits the porphyrin ring of the absorbed heme resulting in the release of free iron ions [ 130 ].
Free iron ions from the small intestine are absorbed by DMT1 (divalent metal transporter 1) in the Fe 2+ form [ 131 ]. However, most dietary non-heme iron is in the Fe 3+ form. The reduction of Fe 3+ to Fe 2+ happens by dietary antioxidants (i.e., ascorbate) [ 122 ] and by luminal ferrireductases such as cytochrome B (CYBRD1, also known as DCYTB) [ 132 ]. Upon cellular entry, iron binds to ferritin. Storage of iron within the cell is mediated by ferritin. It is composed of ferritin light (FTL) and heavy chain (FTH1) [ 133 ].
Efflux of iron ions is allowed by ferroportin (also known as FPN-1, IREG1, MTP1 and Slc40a1 [solute carrier family 4, member 1]), the only known exporter of iron from cells [ 134 ]. After efflux, iron is oxidised (Fe 2+ → Fe 3+ ) by hephaestin or ceruloplasmin in order to be loaded onto transferrin [ 135 ]. Transport of iron within the body is facilitated by transferrin. Transferrin bound iron is non-reactive/non-toxic unlike free iron irons. Cells salvage transferrin molecules by TFR1 (transferrin receptor 1) via receptor-mediated endocytosis [ 136 ].
Further explanation of the iron metabolism and its regulations is out of the scope of this review, but we can recommend reading Review on iron and its importance for human health by Abbaspour N. et al. [ 137 ]. General overview of iron metabolism is drawn in the Fig. 3 .
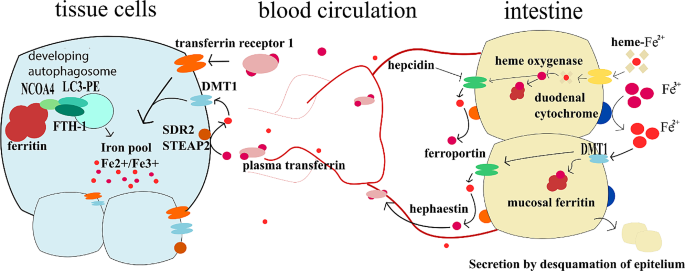
Iron metabolism. This figure summarises the main events in the iron metabolism. In small intestine lumen, iron is found in heme or ionic form. Heme-bound-Fe 2+ enters the enterocyte via a heme transporter and is exposed to heme oxygenase, which frees the iron from heme and oxidises it to the Fe 3+ form. Free Fe 3+ in the intestinal lumen is reduced to Fe 2+ thanks to the duodenal cytochrome B or dietary antioxidants. Fe 2+ can enter the enterocyte through DMT1 (Divalent metal transporter 1). In enterocytes, iron can be stored in mucosal ferritin, or it can be effluxed into blood through ferroportin which is regulated by hepcidin. Once effluxed, Fe 2+ is oxidised to Fe 3+ by hephaestin or ceruloplasmin and is transported bound to plasma transferrin. Very little amounts of iron are transported in the free form. On the site of tissue cells, plasma transferrin binds to transferrin receptor 1 and enters the cells. Tissues can also uptake Fe 2+ iron through DMT1. However, Fe 3+ from the blood first needs to be converted to Fe 2+ by SDR2 or STEAP2 on cells membranes
Following the discovery that O 2 was a common cellular metabolite, it was quickly recognized that the complex of Haber – Weiss reaction could lead to generations of toxic radicals called reactive oxygen species (ROS) [ 138 ]. However, thanks to the research, we now understand that ROS are also essential for physiological cell function and signalling [ 138 ].
Haber - Weiss reaction:
Fenton reaction, although only one part of the Haber - Weiss reaction, is probably the most important reaction of the ROS metabolism. It demonstrates that Fe 2+ has a more potent impact than Fe 3+ [ 139 ].
Fenton reaction:
Iron is a key player in ferroptosis. However, it also plays a significant role in pyroptosis. This connection is also highlighted by the fact that macrophages play a crucial role in iron metabolism [ 140 ]. Therefore, the role of iron in pyroptosis is definitively a field with a significant research potential.
Iron in ferroptosis
There are several assets connecting iron to ferroptosis, present on multiple levels of the process. Firstly, ROS production is mediated by iron via two main pathways. Either directly via Fenton reaction, or indirectly as a cofactor of lipoxygenase (LOX), enabling the enzyme oxidation of PUFAs [ 15 , 141 , 142 ]. Especially, ACSL4 (Long-chain-fatty-acid—CoA ligase 4) and the lipoxygenases 15/15B were found to be pivotal for ferroptosis induced by iron and PUFA dyshomeostatis in dopaminergic neurons [ 10 , 143 ]. Moreover, Glutathione (GSH), a cysteine containing tripeptide, helps to regulate cellular iron levels, iron transport, biosynthesis of iron-binding cofactors, and the formation of iron-GSH complexes [ 144 ]. GSH is needed in regeneration of the GPX4 pathway. Lower levels of glutathione and therefore lower activity of GPX4 leads to higher ROS levels and incidence of ferroptosis [ 145 ]. This is supported by the linkage between impaired expression of cystine/glutamate antiporter, which is essential for GPX4 regeneration, and the resulting iron dyshomeostasis manifested as an increase of ferritin and lipid peroxidation in cerebrospinal fluid. These results also indicate the involvement of ferroptosis in the development of Alzheimer’s disease [ 146 , 147 , 148 ].
Also, heme oxygenase 1 (HO-1) is an important ferroptosis modulating enzyme [ 149 , 150 ]. In hepatocytes, excessive HO-1 expression leads to an iron overload and ferroptosis and interestingly, also to fibroblast growth factor 21 (FGF21) expression [ 148 ]. Notably, FGF21 inhibits HO-1 and thus acts as a ferroptosis suppressor [ 148 ]. HO-1 was also found to modulate ferroptosis in macrophages by regulating iron and ROS levels in response to Bacillus Calmette-Guerin infection [ 151 ].
p53 phosphorylation also occurs in iron overload. In the study by Zhang, P. et al., this process engaged ferroptosis, which promoted the occurrence of apoptosis [ 152 ]. Interestingly, in this study the elevation of p53 phosphorylation did not correspond to MAPK signalling pathway activation [ 152 ]. On the complex role of p53 in ferroptosis, we recommend a review by Yanging Liu and his colleagues [ 153 ].
Ferritinophagy, the autophagic turnover of ferritin, is mediated by a selective cargo receptor Nuclear receptor coactivator 4 (NCOA4) [ 154 ]. NCOA4 binds to phosphatidyl-ethanolamine conjugated light chain 3 microtubule associated protein 1 (MAP1-LC3-PE) and to FTH1 both found on the developing autophagosome membrane. This leads to the exposition of ferritin into autophagosomes [ 155 ]. Knockdown of NCOA4 and autophagy related proteins (ATGs) supresses ferritin degradation, iron accumulation and lipid peroxidation that would otherwise result in ferroptosis [ 156 , 157 ]. This is further supported by the study where overexpression of mitochondrial ferritin inhibited erastininduced ferroptosis [ 157 ]. Based on this evidence, it seems that NCOA4 is related to Xc- system [ 158 ]. Belleli and his team stated that these findings provide genetic evidence of ferroptosis being a process of selective autophagic cell death [ 159 ]. This is further supported by the possibility to induce ferroptosis by ferritinophagy in multiple cancer cell lines [ 158 ]. On the topic of autophagy and ferroptosis, we recommend a review: Ferritinophagy, a form of autophagic ferroptosis: New insights into cancer treatment [ 160 ].
Iron in pyroptosis
Iron relates to pyroptosis via ROS generation [ 44 ]. ROS may function as cellular signalling molecules, which also may alter pyroptosis. In this matter, two pathways have been identified: ROS-Tom20-Bax-Caspase3-GSDME (Tom20 – translocase of the outer membrane) [ 45 ] and ROS-NLRP3-ASC-Caspase-1 (ASC - Apoptosis-associated speck-like protein containing a CARD) [ 161 ].
In melanoma, cell iron generated ROS can induce pyroptosis [ 45 ]. ROS causes oxidation and oligomerization of the mitochondrial outer membrane protein Tom20, which recruits Bax to mitochondria. Bax then facilitates cytochrome c release into cytosol activating Caspase-3. Caspase-3 subsequently induces gasdermine E (GSDME) cleavage, which triggers pyroptosis. Since melanoma cells express a high level of GSDME, the iron-ROS-Tom20-Bax-Caspase-GSDME pathway could be a potential target for melanoma therapy [ 45 ].
Iron as a source of ROS can induce the NLRP3 inflammasome [ 161 ]. The inflammasome subsequently activates ASC and Caspase-1 leading to pyroptosis. A study suggests that neferine, a bisbenzylisoquinoline alkaloid found in lotus ( Nelumbo nucifera ), reduces ROS. Treatment with this alkaloid in endothelial cells inhibited pyroptosis induced by lipopolysaccharide-adenosine triphosphate (LPS-ATP) [ 161 ].
Zinc is the second most abundant TM after iron [ 162 ]. Physiologically, zinc is mostly bound to proteins and small chelators [ 163 ]. The remaining free Zn 2+ pool is strictly controlled and always maintained. Transport of zinc into the cytosol is mediated by 14 ZIPs (Zrt- and Irt-like proteins) [ 164 ]. Export is enabled through 10 ZnT (zinc transporter) proteins [ 165 ]. Roles of zinc in biology are abundant. The most prominent, in terms of cell death modalities, are described below.
Zinc present in domains of metalloproteins enables interactions with DNA, RNA, other proteins or lipids. Furthermore, zinc can also bind subunits to control the assembly of quaternary (same protein) or quinary (different proteins) structure [ 166 ]. Metallothioneins (MT), a protein family of zinc metalloproteins, allow the control of zinc pool. MT’s zinc binding sites differ in affinities ranging over two orders of magnitude [ 167 ] enabling a delicate zinc buffering capacity [ 168 ]. In context of oxidative stress, MT can also react with superoxide and hydroxyl radicals and therefore could provide protection against oxidative stress [ 169 ]. Zinc has a unique role in redox balance as well. In vivo, zinc is only present in the Zn 2+ state and therefore cannot directly impact redox balance [ 170 ]. However, zinc can form a coordinate bond with the thiolate group of cysteine. This zinc thiolate coordination environments are redox-active 166 , hence the sulphur in it can be oxidized [ 171 ]. This fact links zinc to redox and free radical biology, because the number of free thiol groups depends on the oxidative stress [ 171 ]. This mechanism also allows zinc to be released as a second messenger [ 172 , 173 , 174 ]. Such behaviour is shown in the Fig. 4 .

Zinc sulphur complexes. In a reducing environment, Zn 2+ forms complexes with the thiolate groups of cysteine. When this complex is oxidised, cysteines form a disulphuric bond, resulting in the elimination of the coordinate bond and release of Zn 2+ . Once released into the cytoplasm, Zn 2+ can also act as a second messenger
Zinc is regarded as an antioxidant [ 164 ]. However, the term “antioxidant” is misleading since it defines a reagent with a direct substrate reduction ability and zinc does not fit this definition exactly. In biological systems, both the lack and the excess of zinc causes a pro-oxidant state, meaning that both addition and removal of zinc could balance the overall pool. The newly established balance overcomes the pro-oxidant state. Simplistically, zinc cancels pro-oxidant state, like an antioxidant. To differentiate between zinc and true antioxidants, a new term for zinc was proposed: pro-antioxidant [ 175 ].
Redox paradox is a term coined to describe the fact that dietary zinc has different effects than cellular zinc [ 175 ]. Excessive zinc in diet lowers copper uptake, therefore handicapping Cu/Zn SOD (superoxide dismutase), an important antioxidative enzyme [ 175 ]. In conclusion, excessive dietary zinc has a prooxidant effect [ 175 ]. Interestingly, zinc is also a selenium antagonist [ 176 ]. Selenium also exhibits an anticarcinogenic effect by exhibiting antioxidant properties [ 176 ]. Therefore, excessive amounts of zinc abolish the anticarcinogenic effect of selenium [ 177 ].
In the development of both ferroptosis and pyroptosis, zinc may enhance or supress these processes. This phenomenon could only be explained by the complex nature of this element in biology.
Zinc in ferroptosis
The paradoxical nature of zinc regarding redox processes also applies in its relation to ferroptosis. Zinc seems to allow ferroptosis through ZIP7 and may contribute to both ROS generation and ferroptosis through oxidative enzymes [ 178 ]. On the other hand, zinc promotes the degradation of oxidative stress mediators [ 179 ].
In a study by Chen, P. et al., ZIP7 was found as a novel determinant of ferroptosis in breast and renal cancer cells [ 178 ]. ZIP7 controls zinc transport from the endoplasmic reticulum (ER) to the cytosol. Both genetic and chemical inhibition of ZIP7 have been shown to protect against ferroptosis [ 178 ]. This is further supported by the fact that addition of zinc promotes ferroptosis, while the zinc removal by a chelator supresses it [ 178 ]. There is a discussion about zinc affecting phospholipase A2 [ 180 ], lipoxygenase [ 181 ] or xanthine oxidase [ 182 ]. These enzymes could possibly contribute to ROS generation and the incidence of ferroptosis.
During spinal cord injury, zinc in neurons triggers the degradation of oxidative stress products through the NRF2/HO-1 and GPX4 signalling pathways. GPX4 lowers the levels of lipid peroxides, malondialdehyde (MDA) and ROS. Therefore, it inhibits ferroptosis [ 179 ].
Zinc in pyroptosis
The ambivalent behaviour of zinc applies to pyroptosis as well. Oral zinc supplementation during infection supports the NLRP3-ASC-Caspase-1 pyroptotic axis [ 183 ], while zinc gluconate exposure also seems to upregulate NLRP3 and pyroptosis [ 11 ]. However, Zinc finger E-Box binding homeobox 2 (ZEB2) seems to alleviate pyroptosis, while Canonical transient receptor potential-6 (TRPC6) maintains zinc influx and blocks the NLRP3-ASC-Caspase-1 pyroptotic axis [ 184 ].
Oral zinc supplementation in young pigeons challenged with Salmonella enterica serovar Typhimurium reduces bacterial load in the liver, improves NLRP3 protein expression, and tends to increase Caspase-1 protein abundance in the jejunum [ 183 ]. Oral zinc supplementation also enhances the immune response, as well as activates Caspase-1 dependent cell pyroptosis pathways [ 183 ]. Similarly, zinc gluconate exposure results in an increase in the protein levels of NLRP3 and IL-1β in rodent olfactory neuron cell line Odora. This indicates that zinc exposure may lead to pyroptosis [ 11 ].
ZEB2 is a regulator of astrogliosis following ischemia or reperfusion injury [ 184 ]. ZEB2 promotes neuronal proliferation and regeneration by decreasing pyroptosis [ 184 ]. This finding was also verified on a rodent model, where overexpression of ZEB2 promoted astrogliosis, resulted in decreased infarct volume and improved recovery of neurological function by alleviating pyroptosis [ 184 ]. In other study by Shen, B. et al., the authors investigated the role of TRPC6 (Transient receptor potential cation channel) in pyroptosis of renal tubular epithelial cells [ 47 ]. The results show that I/R injury causes downregulation of TRPC6 both in vivo and in vitro [ 47 ]. In the OGD/R (Oxygen-Glucose Deprivation and Reoxygenation) cell model, the inhibitor of TRPC6 (SAR7334) reduced zinc ion influx, aggravated cell death, and upregulated pyroptosis-related proteins. TRPC6 inhibition exacerbated tissue damage and upregulated NLRP3, ASC, Caspase-1, IL-18, and IL-1β in the I/R injury in murine model, which could be alleviated by the administration of ZnCl 2 [ 47 ]. The pyroptosis phenotype could be alleviated by ZnCl 2 and intensified by zinc ion chelator [ 47 ]. Overexpression of A20 reduced the expression of pyroptosisrelated proteins, while A20 deficiency impaired the protective effect of zinc ion [ 47 ].
Human body converts selenium into various compounds like methylselenol or selenocysteine (Sec) [ 185 ]. Selenoproteins play an important role in antioxidant defence, formation of thyroid hormones, DNA synthesis, fertility and reproduction [ 185 ]. Hence, selenium is an essential element. Even though Se is a nonmetal element, it fulfils a special role in relationship with both ferroptosis and pyroptosis. While transition metals tend to induce necrotic cell death modalities, selenium has a protective effect (see further). In ferroptosis, selenium plays its role as a part of GPX4 [ 186 ]. In pyroptosis, lower selenium levels lead to a higher NLRP3 inflammasome activity [ 187 ]. However, the exact mechanism of this interaction is still unclear.
For more detailed information on selenoproteins biochemistry, especially their synthesis, transcription and evolutionary context, we recommend a review by Marcus Conrad and Bettina Proneth [ 188 ].
Selenium in ferroptosis
Selenium has a protective effect and acts against ferroptosis on multiple levels. GPX4 is one of 25 selenoproteins in human body. It directly regulates ferroptosis in a suppressive manner [ 189 , 190 ]. Sec presence instead of Cys in GPX4 is not essential for mice embryogenesis. However, it is essential for neuronal differentiation. This selective mechanism is based on the suppression of peroxideinduced ferroptosis [ 188 ]. Different tissues sensitivity to ferroptosis could be explained by the respective free pool of selenium. Higher pool of selenium increases the function of GPX4 and lowers the sensitivity to ferroptosis [ 191 ]. The proposed selenium-GPX4-ferroptosis axis has a central role in homeostasis of follicular helper T-cells [ 192 ]. Therefore, supplementary selenium can enhance the reaction of body to infections [ 192 ]. Fradejas, N. et al. found that isopentenyltransferase 1 (TRIT1) can isopetenylate SectRNA [ 193 ]. This interference could be new potential approach to downregulate GPX4 expression. This was supported and observed in study with FIN56 (specific ferroptosis inducer) treatment [ 194 ].
Ferroptosis stimulates transcription of protective selenoproteins [ 191 ]. Especially Sp1 (specificity protein) DNA binding is significantly induced by ferroptosis related oxidative stress [ 195 ]. However, this response seems inadequate due to the relative lack of selenium in high demand conditions [ 191 ].
High selenium levels prevent ferroptosis and ferroptosis-independent cell death modalities via the increased transcriptional response of TFAP2c (Transcription factor AP-2 gamma) and Sp1 [ 191 ]. Both indirect and direct delivery of selenium to cerebral ventricle improved the functional recovery after stroke by inhibiting ferroptosis via the mentioned axis [ 191 ]. Tat-SelPep (another Sec containing peptide) also improved outcomes after haemorrhagic stroke in mice [ 191 , 196 ].
Selenium possibly modulates the Nrf2 (Nuclear factor erythroid 2-related factor 2)/GPX4 signalling pathway resulting in ferroptosis inhibition. The effect was tested on BTBR murine model (Autism spectrum disorders model, BTBR T + Itpr3 tf /J ), where it also exhibited a beneficial effect on autismrelevant behaviour [ 197 ].
Ebselen is an organoselenium compound and serves as a pharmacological GPX mimetic [ 196 ]. It exhibits a hydroperoxide-reducing activity like GPX. In vivo experiment confirmed that ebselen stays intact and does not provide selenium for GPX4 [ 196 ]. Ebselen itself was found to be able to prevent ferroptosis in neurons [ 198 ].
Selenium in pyroptosis
Selenium protects against pyroptosis as a part of GPX4 [ 199 ], by altering the PI3K/AKT/PTEN axis [ 200 ], by inhibiting the NLRP3 inflammasome, and of course through its antioxidant properties [ 201 ]. Selenium is utilised by GPX4, which attenuates cadmium-induced ferroptosis and pyroptosis in sheep kidney [ 200 ]. Selenium also antagonises Cd-induced pyroptosis, autophagy and apoptosis by altering the PI3K/AKT/PTEN signalling in heart [ 200 ]. Yeast selenium, one of the most effective organic selenium compounds [ 202 ], exhibits an antioxidant effect on chicken liver, counteracting the cadmium induced pyroptosis [ 203 ]. A selenium-modified phytosomal tripterine was found to reduce cytotoxicity and inflammation by inhibiting the NLRP3 inflammasome and pyroptosis [ 201 ].
On the other hand, Se deficiency itself can lead to pyroptosis via ROS-NLRP3-IL-1β axis [ 204 ]. Also, during Se depletion, both TXNRD3 (Thioredoxin Reductase 3) [ 205 ] and miR-1656 target NLRP3 activation and pyroptosis [ 48 ]. In pig spleen, selenium deficiency causes pyroptosis via ROS/NLRP3/IL-1β signalling pathway [ 204 ]. A selenoprotein thioredoxin reductase 3 (TXNRD3) was found to be associated with pyroptosis and necrosis. In TXNRD3 knock-out mice the expression of NLRP3, Caspase-1, RIPK3 (Receptor-interacting serine/threonine-protein kinase 1), and MLKL (Mixed Lineage Kinase Domain-Like Pseudokinase) increased significantly [ 205 ]. However, overexpression of TXNRD3 leads to calcium outflow-induced oxidative stress followed by necrosis and pyroptosis [ 205 ]. A micro-RNA miR-1656 targets GPX4 leading to NLRP3 activation and pyroptosis in selenium deficient broiler kidney tissues [ 48 ].
In vivo, copper exists in two stable ion forms (Cu + and Cu 2+ ), making redox reactions possible [ 206 ]. Therefore, the maintenance of copper homeostasis is crucial [ 207 ]. Cellular influx of copper is mediated via the Cu transporter 1 (CTR1) [ 208 , 209 ]. While Cu-ATPases (ATP7A and ATP7B) facilitate efflux of copper [ 210 ]. Intracellular copper is either chelated by metallothionein, as storage, or bound to Cu chaperones [ 211 ]. These chaperones also serve as transporters - taking copper ions to their targets [ 210 ]. Many oxido-reductases require copper to function properly. The notable ones are Cu/ZnSOD, ceruloplasmin, cytochrome c oxidase, lysyl oxidase, tyrosinase and dopaminebetahydroxylase [ 212 ]. Copper also impacts iron homeostasis via ferroxidases haephaestin and ceruloplasmin [ 213 ]. These copper-rich enzymes, each containing 6 atoms of copper, are essential for electron transfer allowing ferroxidase activity [ 214 ].
Copper is proven to be related to both ferroptosis and pyroptosis. In the case of copper in ferroptosis, there is a common mechanism found in most TMs – the disruption of iron homeostasis. However, in this case the redox activity of copper itself should not be overlooked.
Copper in ferroptosis and pyroptosis
Copper induces the autophagic degradation of GPX4 resulting in ferroptosis [ 215 ]. On the other hand, copper depletion enhances mitochondrial perturbation. This process depletes the antioxidative mechanism leading to ferroptosis as well [ 216 ]. Cuprizone is a synthetic copper chelator. In experimental animals, it is used to cause demyelination [ 217 ]. Jhelum P. et al. proved that cuprizone diet-induced destruction of oligodendrocytes is caused by ferroptosis [ 217 ]. They showed that chelating copper leads to the expression of molecules rapidly mobilizing iron from ferritin. The released iron then shall trigger iron-mediated lipid peroxidation resulting in ferroptosis [ 217 ]. To conclude, it seems both copper overload and depletion lead to ferroptosis. This highlights the importance of copper homeostasis.
Copper induces pyroptosis in pig jejunal epithelial cells. Copper excess causes endoplasmic reticulum stress via IRE1α-XBP1 (Inositol-requiring enzyme 1, X-box binding protein spliced) pathway and this pathway subsequently mediates pyroptosis [ 49 ].
Recently, Peter Tsvetkov and his team distinguished a new copper mediated cell death modality cuproptosis [ 49 , 218 ]. In this case, copper binds directly to lipoylated components of the tricarboxylic acid cycle causing their aggregation. The subsequent loss of iron-sulphur cluster proteins leads to proteotoxic stress and cell death [ 49 , 218 ].
Molybdenum (Mo) is a vital micronutrient that acts as an enzyme cofactor. In humans, 4 enzymes are known to contain Mo: xanthine oxidase, sulphite oxidase, aldehyde oxidase and mitochondrial amidoxime-reducing component (mARC) [ 219 , 220 ]. Once in body, Mo primarily accumulates in the liver and kidneys. The kidneys are particularly prone to Mo toxicity, as approximately 50% of this element is metabolised there. Excessive Mo intake has been linked to chronic renal failure [ 221 ], poor growth, anaemia, diarrhoea, significant functional and morphological damage in several organs, and even death [ 222 ].
Mo compounds can catalyse ROS generation [ 219 ]. This fact links them to ferroptosis, but also to possible cancer treatment [ 219 ]. However, the role of this TM is unclear in pyroptosis. Up to date published results were obtained in co-induction with cadmium (Cd) [ 50 , 223 , 224 ]. Therefore, the role of Mo in these cases, as well as the interaction with Cd, is unclear.
Molybdenum in ferroptosis
MoO 4 2− as a catalyst can turn hydrogen peroxide H 2 O 2 (a common ROS in malignant tumours) to singlet oxygen ( 1 O 2 ). However, as authors of this recent study state, molybdate ions are not suitable for redox-based cancer treatments [ 219 ]. Their study presents the molybdenum sulphide (MoB) nanocatalysts. MoB simultaneously generates 1 O 2 and superoxide anions (O 2 • − ) from H 2 O 2 . Interestingly, MoB-created ROS induce lipid peroxidation and result in ferroptosis. Hence MoB could present a possible efficient cancer therapy [ 219 ].
Molybdenum in pyroptosis
Molybdenum overexposure can lead to oxidative stress and pyroptosis [ 50 ]. Moreover, Mo induces autophagy in the kidneys as well [ 225 ]. Mo and Cd co-induce pyroptosis in duck brains. The mechanism involves the inhibition of the NRF2-mediated antioxidant defence response [ 223 ]. Other possible mechanism is PTEN/PI3K/AKT axis found in Mo and Cd co-induced pyroptosis and apoptosis in the livers of Shaoxing ducks ( Anas platyrhynchos ) [ 224 ].
Although cobalt is an essential element as the metal core of vitamin B12, excessive cobalt (Co) exposure has various adverse health effects [ 226 ]. Cobalt occurs in alloys, batteries and also in artificial joints, from where it can be up-taken by a human body [ 226 , 227 ].
Cobalt induces ferroptosis via a Fenton-like reaction [ 228 ] and pyroptosis via ROS-NLRP3-Caspase-1 pathway [ 229 ]. The same effect is induced by cobalt nanoparticles, which we mentioned here due to their clinical importance [ 227 ]. These nanoparticles are the result of the wear of artificial joints [ 227 ]. However, other TM nanoparticles are out of scope of our review.
Cobalt in ferroptosis
Co ions in water produce ROS in a Fenton-like reaction. Free Co + ions can generate \(\:\text{O}{\text{H}}^{{\bullet\:}}\:\) radicals [ 228 ].
On the other hand, the reaction of free Co 2+ with H 2 O 2 does not generate any significant amount of \(\:\text{O}{\text{H}}^{{\bullet\:}}\) radicals. However, biological chelators such as GSH or beta-ananyl-3-methyl-L-histidine alter the oxidation-reduction potential of Co 2+ enabling another Fenton like reaction [ 228 ].
Supporting these claims, Co particles from endoprostheses increase ROS, Fe 2+ levels, lipid peroxidation, GSH consumption and inhibit GPX4 activity [ 227 ]. These processes induce a ferroptosis-like cell death. Importantly, α-lipoic acid (ALA), a natural antioxidant, a scavenger of free radicals and a chelator of toxic metals, can efficiently counteract the effects of Co particles [ 227 ].
Cobalt in pyroptosis
Wear particles from implant surfaces induce periprosthetic osteolysis. A study by Xue S. et al., focuses on cobalt-chromium-molybdenum implant wear particles (CoPs) [ 229 ]. They found that CoPs exposure and accumulation in macrophages induces ROS generation and subsequently NLRP3dependent pyroptosis [ 229 ]. This, in turn, also stimulates the release of pro-inflammatory cytokines such as IL-18, IL-1β, and HMGB1 (High-mobility group box 1). CoPs also led to mitochondrial damage in macrophages, accelerating ROS production and NLRP3-dependent pyroptosis [ 229 ].
Cobalt can induce acute kidney injury (AKI) through hypoxia-reoxygenation injury (HRI) [ 230 ]. Liu, W. et al., induced HRI-AKI by CoCl 2 both in vitro and in vivo [ 230 ]. According to their study, GSDMEmediated pyroptosis was involved in cell damage under HRI-AKI. GSDME is cleaved by Caspase-3/8/9 leading to pyroptosis [ 230 ]. Autophagy also occurs in HRI-AKI. In this case autophagy also induces a GSDME-mediated pyroptosis via apoptotic pathways [ 230 ].
Nickel (Ni) has a dual nature of an essential as well as a toxic element [ 231 ]. In higher organisms, a higher perinatal mortality, changes in grooming behaviour, and a decreased growth are also present in the absence of Ni [ 231 ]. Nickel depletion, although rare, reduces intra-uterine development and iron adsorption leading to anaemia in humans [ 231 ]. Ni is associated with allergies, carcinogenesis and infectious agents, which rely on nickel-based enzymes [ 232 ]. However, there is little evidence of Ni accumulating in the food chain. Moreover, Ni, unlike Pb, is not a cumulative toxin for humans. Almost all cases of acute poisoning are due to nickel carbonyl [ 233 ].
Nickel in ferroptosis
In mice, Ni causes histopathological alterations of liver and an increase in AST (aspartate aminotransferase) and ALT (alanine aminotransferase) serum levels [ 234 ]. There is also an increase in MDA (Malondialdehyde) production, while total antioxidant capacity and GSH content are reduced [ 234 ]. Then ROS levels increase, and mitochondrial membrane depolarizes. Meanwhile, Ni increases iron content, upregulates cyclooxygenase 2 and down-regulates GPX4, FTH1 and NCOA4 resulting in ferroptosis [ 234 ].
Nickel in pyroptosis
Selenoprotein M (SelM) is a common antioxidant protein found in various tissues [ 235 ]. In SelM knockout mice’ spleen, Ni induces the expression of ASC, AIM2, NLRP3, Caspase-1, IL-18 and IL-1β and results in pyroptosis [ 236 ]. Interestingly, in the same experiment, melanin was found to alleviate the impact of Ni exposure and offered protection against pyroptosis [ 236 ].
Elemental Platinum is very biologically inert and therefore of a relatively small interest as a part of a metabolism. However, its compounds are used as common chemotherapeutics and exhibit a variety of interesting properties. This is also the field, where the connection between Pt and ferroptosis and pyroptosis is established. A well-written platinum (Pt) biochemistry review regarding Pt coordination complexes and Pt-DNA interaction was published by Douple and Richmond [ 237 ]. Generally, the toxicity of noble metals such as Pt is still unclear [ 238 ].
Platinum in ferroptosis
Cis-platin administration induces ferroptosis in multiple ways. Cis-platin was found to inactivate GPX and deplete GSH in A549 and HCT116 cells resulting in ferroptosis [ 239 ]. Furthermore, cis-platin increases the expression of both transferrin receptor and ferritin, which results in increased cellular iron levels [ 240 ]. Cis-platin treatment was followed by an increased expression of ferroptosis markers, COX2 (cyclooxygenase-2) and 4hydroxynonenal (4-HNE) and ferroptosis respectively [ 240 ]. Ferrostatin-1 or VPA (valproic acid) decreases the occurrence of ferroptotic phenotype after cis-platin administration [ 240 , 241 ]. Thus, cisplatin treatment together with ferroptosis induction makes a promising strategy for ovarian cancer [ 242 ].
Interestingly, the activation of vitamin D receptor seems to have a protective effect in cis-platin induced ferroptosis [ 243 ]. Please note, that cis-platin also induces other cell death modalities, i.e., apoptosis and necrosis [ 244 ].
Colorectal cancer cells are often resistant to oxaliplatin treatment, a cis-platin derived drug [ 245 ]. This resistance can be reversed by ferroptosis induction, possibly by the mechanisms of lipid peroxidation, and iron metabolism disruption [ 246 ]. Oxaliplatin sensitivity depends on the KIF20A-NUAK1 (Kinesin family member 20 A, SNF1-like kinase 1) and GSK3β-NRF2 (glycogen synthase kinase 3β, nuclear factor erythroid 2-related factor 2) signalling pathways in colorectal cancer cells [ 247 ].
Novel cis-platin derived drugs are being synthetized. Their properties are often targeted to evade side effects or to overcome resistance in cancer cells. For example, platinum(IV) complexes conjugated with ligustrazine-based chalcones showed promising anticancer effects by inducing apoptosis and ferroptosis through accumulation of LPOs and inhibiting xCT-GPX4 axial pathway [ 248 ]. Platin B is another drug derived from cis-platin by addition of 4-carboxylphenylboronic acid [ 249 ]. This acid is a powerful GSH scavenger [ 249 ]. Therefore, the administration of Platin B leads to cellular GSH depletion, which triggers ferroptosis [ 249 ].
Platinum in pyroptosis
Cis-platin can induce pyroptosis in tumour cell in many possible mechanisms. In triple negative breast cancer cells, cis-platin upregulates the long non-coding RNA maternally expressed gene 3 (MEG3) [ 51 ]. This subsequently activates the MEG3-NLRP3-Caspase-1-GSDMD pyroptotic axis [ 51 ]. Other study confirms that the cleavage GSDMD after cis-platin administration induces acute kidney injury by pyroptosis [ 52 ].
Furthermore, in oesophageal cancer cells cis-platin targets calpain-1 (CAPN1) and calpain-2 (CAPN2) [ 53 ]. In this particular study, high expression of CAPN-1 and CAPN-2 was associated with favourable clinical outcomes after cis-platin treatment [ 53 ]. In this particular case, scientists discovered that cis-platin induces the CAPN1/CAPN2-BAK/BAX-Caspase-9-Caspase-3-GSDME pathway [ 53 ]. Caspase-3GDSME activation was also described in another chemotherapeutic agent paclitaxel [ 54 ]. However, cis-platin seems to be a stronger inductor of pyroptosis than paclitaxel [ 54 ].
Interestingly, cis-platin exhibits a protective effect by inhibiting Caspase-3-mediated GSDME-derived pyroptosis in noncancerous tissues of squamous cell carcinoma patients [ 250 ].
Lobaplatin induces pyroptosis by GSDME cleavage and Caspase-3 activation in colon cancer cells [ 55 ]. Knock-out of GSDME results in a switch from pyroptosis to apoptosis. Lobaplatin treatment increases ROS levels and JNK (c-Jun N-terminal kinase) phosphorylation, which can be reversed by ROS scavenger NAC (Nacetylcysteine) [ 55 ]. JNK activation recruits Bax to mitochondria, resulting in cytochrome c release to cytosol, followed by Caspase-3/9 cleavage and pyroptosis [ 55 ]. Lobaplatin induces Caspase-3-GSDME pyroptosis also in cervical cancer cells [ 56 ]. Lobaplatin can also induce pyroptosis by degradation of cell inhibitor of apoptosis protein-1/2 (cIAP1/2) in nasopharyngeal carcinoma cells [ 57 ]. While the recovery of cIAP1/2 inhibits pyroptosis. Unsurprisingly, the inhibition of cIAP1/2 by a specific antagonist dramatically enhanced lobaplatin induced pyroptosis [ 57 ]. However, the inhibition of ripoptosome (RIPK1/Caspase-8/FADD), of ROS and Caspase-3 cleavage cancels the before mentioned synergistic effect [ 57 ].
Targeting the cyclic GMP-AMP synthase-stimulator of the interferon gene (cGAS-STING) pathway is a potent anticancer immunotherapeutic strategy [ 251 ]. Ling, Y. et al. developed two PtII complexes (Pt1 and Pt2), which photoactivate the cGAS-STING pathway, while also inducing pyroptosis in cancer cells. This process triggers a potent anticancer immune response both in vitro and in vivo [ 251 ].
Cadmium (Cd) is a non-essential toxic heavy metal [ 252 ]. Chronic exposure leads to systemic toxicity and causes various cancers (mostly in the lung, prostate, breast, pancreas, nasopharynx and kidney) [ 252 ]. Cadmium’s long biological half-life (25–30 years) leads to accumulation in the human body for decades [ 253 ]. Interestingly, a corelation was found between prostate cancer progression and rising Cd serum levels [ 254 ].
Cadmium disrupts protein stability [ 255 ]. This fact connects Cd to both ferroptosis and pyroptosis. However, this is presented in a very high variability of interactions [ 255 ]. Therefore, the specificity of these interactions seems very low. Nevertheless, cadmium still causes these cell death modalities. A better understanding of cadmium-protein interactions is needed, this fact provides a wide area for further research.
Cadmium in ferroptosis
Cd can induce ferroptosis in multiple ways. Cd activates heme oxygenase 1 (HO-1) leading to free iron release from heme [ 256 ]. Endoplasmic reticulum stress-mediated ferritinophagy caused by Cd exposure, also raises cellular free iron [ 257 ]. Interestingly, Cd also lowers testosterone synthesis by promoting ferroptosis and preventing the fusion of autophagosome with lysosome [ 256 , 258 , 259 , 260 , 261 , 262 ].
Cd induced ferroptosis triggers the PERKeIF2α-ATF4-CHOP (RNA-like endoplasmic reticulum kinase, Eukaryotic Initiation Factor 2 alpha, Activating Transcription Factor 4, CCAAT-enhancer-binding protein homologous protein) pathway, corresponding with the endoplasmic reticulum stress theory. It is further supported by the suppression of ferroptosis incidence after ER stress inhibition by iron chelating [ 46 , 257 ].
Cd also causes lipid peroxidation and inhibits the Kelch-like ECH-associated protein 1 (KEAP1)NRF2ARE (antioxidant response element) signalling pathway [ 263 ]. This results in ferroptosis, which also impairs the behaviour and growth of the tested Drosophila [ 263 ].
Cd modulates the miR-34a-5p/Sirt1 axis. In PC12 (rat pheochromocytoma) cells Cd promotes miR-34a-5p to target Sirtuin 1 resulting in cytotoxicity [ 264 ]. Cytotoxicity, as well as both apoptosis and ferroptosis is attenuated by miR-34a-5p knock-out in Cd exposure [ 264 ].
Cd alters the Gpx4/Ager (receptor for advanced glycation endproducts)/p65 axis. In a study on pancreatic β-cells, a treatment with Cd induced ferroptosis and Ager/p65 related inhibition [ 265 ]. Interestingly, ferroptosis inhibitor Fer-1 disrupts the Ager activation and ferroptosis [ 265 ].
Vitamins A, D and E reduce lipid peroxidation, oxidative stress, and also inflammatory responses in Cd exposed MCF7 (Michigan Cancer Foundation-7, breast cancer) cells [ 266 ]. While vitamins A and E are known antioxidants, vitamin D surprisingly works in this case as well. This might indicate a connection between vitamin D, oxidative stress and maybe even ferroptosis.
Cadmium in pyroptosis
Cd contributes to pyroptosis in different manners. Cd exposure results in a dose-dependent increase in ROS generation [ 58 , 267 ]. In duck renal tubular epithelial cells, this results in a percentual increase of pyroptotic cells, lactate dehydrogenase (LDH), NO, IL-18 and IL-1β releases and relative conductivity [ 268 ]. The whole process is accompanied by the upregulation of mRNA for PTEN (phosphatase and tensin homolog), ASC, NLRP3, NEK7 (NIMA-related kinase 7), Caspase-1, GSDMA, GSDME, IL-18 and IL-1β [ 268 ]. With the corresponding increase in protein levels of PTEN, Caspase-1, p20, NLRP3, ASC, GSDMD. While PI3K, AKT and p-AKT expression levels decreased [ 268 ].
At high levels, Cd seems to increase the expression of NLRP3, Caspase-1, IL-1β, and IL-18, while this process can be attenuated by ROS inhibitor NAC [ 58 ]. This supports the fact that the NLRP3 inflammasome and Caspase-1 expression is a common response to pyroptosis in endothelial cells challenged by toxic chemicals, with ROS production playing a central role [ 58 ]. GSDME executes pyroptosis induced by Cd toxicity, ROS generation and NLRP3 activation in a triple-negative breast cancer (TNBC) MDAMB-231 cell model [ 58 ]. From this study it also seems, that low concentrations of Cd result in apoptosis, while high concentrations contribute to pyroptosis accompanied by LDH release. z-VAD, a broad-spectrum Caspase inhibitor, inhibits all the previously mentioned processes [ 58 ]. Additionally, Cd also activates the p38-MAPK signalling pathway, leading to nuclear translocation of NF-κB/p65 (Nuclear factor kappa-light-chain-enhancer of activated B cells) and the expression of proinflammatory cytokines. Interestingly, Cd caused cell cycle arrest in S phase with cyclin alterations [ 58 ]. Subsequently, the expression of pro-inflammatory cytokines is activated, and these are then also contributing to pyroptosis [ 59 ]. Cd activates IRE-1α/XBP-1 (X-box binding protein 1) branch of endoplasmic reticulum stress in human proximal tubular epithelial HK-2 cells. This induces Caspase-1 and NLRP3 inflammasome-dependent pyroptosis, therefore causing nephrotoxicity [ 269 ].
Mercury, lead, uranium
Mercury is a toxic heavy metal. Human exposure mostly results from fish consumption and dental amalgams [ 270 ]. Organic mercury compounds can induce apoptosis and necrosis in a variety of cell types [ 271 ]. However, mercury (Hg 2+ ) and methylmercury (CH 3 Hg + ) exposure results in ferroptosis [ 271 ]. In human embryonic kidney 293T cells, HgCl 2 and CH 3 HgCl trigger ferroptosis, explaining their nephrotoxicity [ 271 ]. Hg 2+ and CH 3 Hg + stress down-regulates GPX4 expression. CH 3 HgCl also directly binds to the selenol group (-SeH) of GPX4 inhibiting its activity [ 271 ]. Remarkably, selenite supplementation enhances GPX4 activity and antagonises the cytotoxicity of CH 3 HgCl [ 271 ].
In LPS treated mice, mercury disrupts mitochondrial ROS production [ 272 ]. This further leads to the inhibition of ASC pyroptosome and GDSMD cleavage, resulting in impaired inflammatory response and attenuated pyroptosis [ 272 ].
Lead (Pb) is a toxic environmental pollutant associated with adverse effects on human health such as neurotoxicity, carcinogenicity, and others [ 273 ]. Pb enhances blood-cerebrospinal fluid barrier (BCSFB) permeability and accumulates in brain tissue, leading to its dysfunction [ 274 ]. Choroid plexus (CP) cells are the main components of the BCSFB crucial to its functions [ 274 ]. Ferroptosis was identified as the main cell death modality in primary cultured CP cells after Pb exposure [ 274 ]. Under these conditions, 16 ferroptosis-related genes show alteration of expression [ 274 ]. Notably, GPX4, Slc7a11, TFR, and Slc40a1 [ 274 ]. Moreover, inhibition of ferroptosis enhances CP cell’s viability and reduces BCSFB permeability [ 274 ].
Pb increases NLRP3 expression and Caspase-1 cleavage, and also increases autophagy and NF-κB phosphorylation [ 275 ].
Uranium (U), a toxic and radioactive metal, presents an environmental and health matter of concern [ 276 ]. Uranium was found to be connected to ferroptosis in two ways. U leads to iron accumulation and a decrease in GPX activity in Vicia faba roots [ 277 ]. These factors trigger ferroptosis like cell death [ 277 ]. The researchers also found that the cell deaths were promoted by addition of Fe, while inhibited by antioxidants (ferrostatin and Vitamin E) and iron chelator ciclopirox olamine [ 277 ].
Researchers investigated the effects of long-term low dose gamma radiation from U tailings on gene expressions in the AHH-1 lymphocytes cells [ 278 ]. It is important to note that gamma radiation itself can generate ROS [ 279 ]. In the experiment, the expression of TFR , SLC3A2 , SLC39A , FTH1 , ACSL4 , and GPX4 genes was found sensitive to low-dose radiation. These genes represent the ferroptotic pathway, hence it is safe to assume connection between gamma radiation and ferroptosis [ 278 ].
Conclusions
In this review, we described the role of transition metals in ferroptosis and pyroptosis. Ferroptosis is a cellular death modality caused by the oxidative stress-induced lipid membrane disruption. The whole process occurs when oxidative stress presented by ROS is greater than the capacity of the cells’ antioxidant mechanisms. The main representants of antioxidant mechanisms, in the case of ferroptosis, are the Xc- system and the GPX4 axis. As the name ferroptosis suggests, ferrous ions are the main cause of oxidative stress, via the Fenton reaction. However, other metals than iron also interact with this pathway. These interactions are presented in 3 main ways:
metals create ROS themselves, or lead to the creation of ROS as enzyme cofactors and by signalling pathway alterations,
metals impair the antioxidant systems (GPX4 axis),
metals affect iron metabolism, increase its availability for the Fenton reaction and result in the ROS formation.
Meaning, some increase the incidence of ferroptosis, while others act in a protective manner (for example Se). Nickel, mercury, lead, and uranium are a group of TMs, which cause ferroptosis by a dysregulation of iron homeostasis. Interestingly, zinc has a dual effect.
To give a better perspective on each metal’s effects a graphics of the above mentioned ferroptosis pathways is presented in this work (Fig. 5 ). Additionally, every metal’s pathway is listed in Table 1 to complement Fig. 5 in a more detailed fashion.
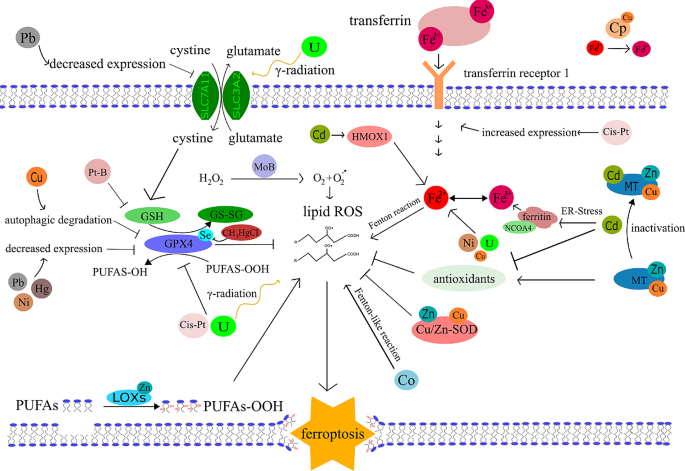
Ferroptosis and significant transition metals overview. In this figure we summarise an overview of main roles of various transition metals in ferroptosis documented in this review. Iron (Fe) generates hydroxyl radicals by undergoing the Fenton reaction. The iron pool is supplemented by NCOA4 mediated ferritinophagy or by iron import through transferrin receptor 1. Zinc (Zn) is found in abundant superoxide dismutase (Cu/Zn-SOD) and in metallothionein (MT). Cu/Zn-SOD scavenges superoxide and MT has a pro-antioxidant effect. Zinc is further present in lipoxygenase (LOX) generating PUFAS-OOH. Selenium (Se) acts as a ferroptosis suppressor mainly by the presence in GPX4. Copper (Cu) is found in Cu/Zn-SOD and MT. Ceruloplasmin (Cp) has a key role in the maintenance of Fe 2+ and Fe 3+ pool balance. Cu was also found to induce an autophagic degradation of GPX4. Molybdenum (Mo) participates in the form of molybdenum sulphate (MoB) on the catalytic generation of superoxide radical. Cobalt (Co) undergoes Fenton-like reaction generating ROS. Nickel (Ni) dysregulates iron homeostasis decreases expression of GPX4. Platinum (Pt) is represented by the platinum derived chemotherapeutics – cis-platin (Cis-Pt) and its derivate Platin B (Pt-B). Cis-platin alone increases expression of transferrin receptor 1 and inhibits GPX4. 4-carboxylphenylboronic acid added in Platin B is potent GSH scavenger which depletes GPX4 from its crucial substrate. Cadmium (Cd) activates Heme oxygenase 1 (HMOX1), inactivates MT and various antioxidants and induces ER stress mediated ferritinophagy. Mercury (Hg) decreases the expression of GPX4. CH3HgCl also directly binds to the selenol group (-SeH) of GPX4 inhibiting its activity. Lead (Pb) decreases expression of GPX4 and SLC7A11 transport system. Uranium (U) dysregulates iron homeostasis and by γ-radiation generates ROS and alters expression of SLC3A2. All the pathways are mentioned in the following summarising Table 1
In conclusion, the influence of metals on ferroptosis is complex and has a lot of implications. The understanding of these processes is crucial for developing successful strategies to treat health issues concerning inflammation, immune response pathology, carcinogenesis, and many others.
Pyroptosis is characterised by the activation of Caspase-1,4,5 or 11 resulting in gasdermin cleavage and pore formation. Originally, this cell death modality was discovered as a response to PAMPs – bacterial toxins. PAMPs can trigger pyroptosis via canonical and non-canonical pathways. After the activation of the canonical pathways (with PAMPs or DAMPs), the inflammasome is created, leading to downstream activation of Caspase-1. In the case of the non-canonical pathway, bacterial endotoxins activate Caspese-4 or -5. Both pathways end in the Caspases-mediated cleavage of GSDMD (or other gasdermins), leading to pore formation and subsequent lysis of the cell. However, both pathways may be significantly altered and even initialized by metal ions. These interactions are following:
metals directly, or via signalling pathways interact with inflammasome formation,
metals induce ROS which contribute to inflammasome activation,
metals activate Caspase-3, which catalyses the non-canonical pathway.
Like in case of ferroptosis, a summarising graphics (Fig. 6 ) depicting the effects of metals on pyroptosis was created. All the relevant pathways are also listed in Table 1 . Notably, Table 1 is no comparison between ferroptosis and pyroptosis, both cell death modalities are unique. This table (Table 1 ) summarises and gives a more detailed legend to Figs. 5 and 6 .
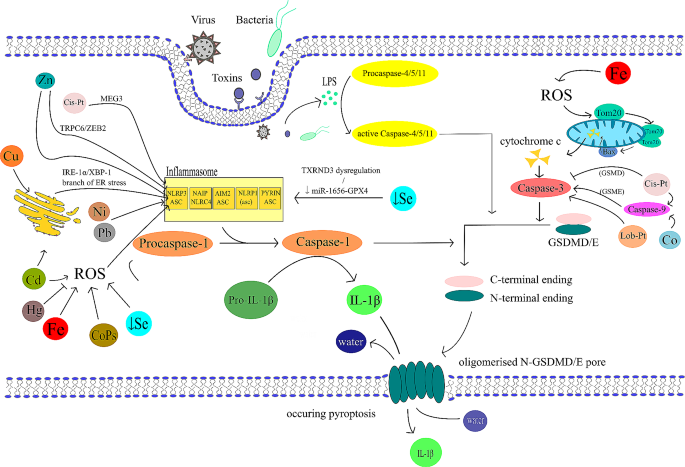
Pyroptosis and significant transition metals overview. In this figure we summarise an overview of main roles of various transition metals in pyroptosis documented in this review. Iron (Fe) generates ROS, which activates ROS-NLRP3-ASC-Caspase-1 or ROS-Tom20-Bax-Caspase-GSDME pathway. Zinc (Zn) contributes to pyroptosis by NLRP3-ASC-Caspase-1 or alleviates it by the TRPC6-NLRP3-ASC-Caspase-1 or ZEB2. Selenium (Se) depletion leads to the increase in free ROS or TXRND3 dysregulation. Se deficiency induces upregulation of miR-1656 leading to heightened expression of pyroptosis associated genes such as NLRP3 by suppressing GPX4 release. Copper (Cu) activates inflammasome by the IRE1α/XBP1(ER stress)-NLRP3 axis. Cobalt (Co) nanoparticles induce the ROS-NLRP3-ASC-Caspase-1 pathway. Cobalt also induces GDSME-Caspase-3/8/9 activation. Nickel (Ni) up-regulates ASC, AIM2, NLRP3, Caspase-1, IL-18 and IL-1β. Platinum (Pt) is represented by the most used platinum derived chemotherapeutics – cis-platin (Cis-Pt) and its derivate lobaplatin (Lob-Pt). Cis-Pt induces the CAPN1/CAPN2-BAK/BAX-Caspase-9-Caspase-3-GSDME pathway. Cis-Pt also activates the MEG3-NLRP3-Caspase-1-GSDMD axis. Cis-Pt inhibits Caspase-3-GSDMD pathway in non-cancerous cells. Lobaplatin activates Caspase-3-GSDME pathway. Cadmium (Cd) activates both ROS-NLRP3-Caspase-1 and IRE1α/XBP1(ER stress)-NLRP3 pathways. Lead (Pb) increases NLRP3 and Caspase-1 expression. Mercury (Hg) disrupts ROS production and inhibits the ASC pyroptosome and GDSMD cleavage. Lead (Pb) increases NLRP3 expression and Caspase-1. Molybdenum (Mo) role in pyroptosis is unclear and therefore we didn´t visualised its role in the scheme. Uranium (U) role in pyroptosis wasn´t yet documented. All the pathways are mentioned in the following summarising Table 1
To conclude, both ferroptosis and pyroptosis are important pathophysiological mechanisms. Transition metals interfere with these cell death modalities. Their homeostasis is crucial in induction both triggering and inhibiting pathways. These findings highlight the importance of TMs in cell biology. Currently, many articles are published on this topic. This promises further clarification of some other regulatory pathways, or even progress in the treatment of serious diseases caused by damage to these cell death modalities regulation together with corrupted metal homeostasis.
Data availability
No datasets were generated or analysed during the current study.
Abbreviations
Tumor necrosis factor, alpha-induced protein 3
Absent in melanoma
Acyl-CoA synthetase long-chain family member
α-lipoic acid
Alanine transaminase
Acute kidney injury
Protein kinase B
Antioxidant response element
Apoptosis associated speck-like protein containing a CARD
Aspartate transaminase
Autophagy related proteins
B-cell lymphoma 2-antagonist killer 1
B-cell lymphoma 2-like protein 4
Blood-cerebrospinal fluid barrier
Cyclic GMP-AMP synthase-stimulator of the interferon gene
Cell inhibitor of apoptosis protein
Cobalt-chromium-molybdenum implant wear particle(s)
Choroid plexus
Cu transporter
Damage associated molecular pattern(s)
Deoxy-ribonucleic acid
Divalent metal transporter
Endoplasmic reticulum
Extracellular signal-regulated kinase
Fibroblast growth factor
Ferroptosis suppressor protein
Ferritin light chain
Ferritin heavy chain
Glutathione peroxidase
Gasdermin(s)
Glutathione
High-mobility group box
Heme oxygenase
Heme oxygenase 1
Hypoxia-reoxygenation injury
Heme responsive gene
Phosphorylated Heat shock protein 1
Interleukine
C-Jun N-terminal kinase
Lactate dehydrogenase
Lipoxygenase
Lipopolysaccharide-adenosine triphosphate
Kelch-like ECH-associated protein
Mitogen-activated protein kinase
Mitochondrial amidoxime-reducing component
Malondialadehyde
Long non-coding RNA maternally expressed gene 3
Messenger ribonucleic acid
Metallothionein
Nuclear receptor coactivator
NIMA-related kinase
Nuclear factor kappa-light-chain-enhancer of activated B cells
Nuclear factor erythroid 2–related factor
Nod like receptor
NLR family CARD domain containing protein
NLR family pyrin domain containing
Pathogen associated molecular pattern(s)
Phosphoinositide 3-kinase
Polyunsaturated fatty acid(s)
Phosphatase and tensin homolog
Ribonucleic acid
Reactive oxygen species
Selenocysteine
Selenoprotein M
Superoxide dismutase
Emphasis Type="Italic”>- Salmonella enterica </Emphasis> serovar Typhimurium
Transcription factor AP-2 gamma
Transferrin receptor
Triple-negative breast cancer
Tumour necrosis factor
Transition metal(s)
Isopentenyltransferase
Canonical transient receptor potential
Thioredoxin reductase
Zinc finger E-Box binding homeobox
Zrt- and Irt-like proteins
Zinc transporter
Dolma S, Lessnick SL, Hahn WC, Stockwell BR. Identification of genotype-selective antitumor agents using synthetic lethal chemical screening in engineered human tumor cells. Cancer Cell. 2003;3(3):285–96.
Article PubMed CAS Google Scholar
Dixon SJ, Lemberg KM, Lamprecht MR, Skouta R, Zaitsev EM, Gleason CE, et al. Ferroptosis: an iron-dependent form of nonapoptotic cell death. Cell. 2012;149(5):1060–72.
Article PubMed PubMed Central CAS Google Scholar
Gao M, Jiang X. To eat or not to eat-the metabolic flavor of ferroptosis. Curr Opin Cell Biol. 2018;51:58–64.
Stockwell BR, Friedmann Angeli JP, Bayir H, Bush AI, Conrad M, Dixon SJ, et al. Ferroptosis: a regulated cell death Nexus linking metabolism, Redox Biology, and Disease. Cell. 2017;171(2):273–85.
Kuang F, Liu J, Tang D, Kang R. Oxidative damage and antioxidant defense in Ferroptosis. Front Cell Dev Biology. 2020;8.
Ward JPT. From physiological Redox Signalling to oxidant stress. Adv Exp Med Biol. 2017;967:335–42.
Chen X, Li J, Kang R, Klionsky DJ, Tang D. Ferroptosis: machinery and regulation. Autophagy. 2021;17(9):2054–81.
Shintoku R, Takigawa Y, Yamada K, Kubota C, Yoshimoto Y, Takeuchi T, et al. Lipoxygenase-mediated generation of lipid peroxides enhances ferroptosis induced by erastin and RSL3. Cancer Sci. 2017;108(11):2187–94.
Yuan H, Li X, Zhang X, Kang R, Tang D. Identification of ACSL4 as a biomarker and contributor of ferroptosis. Biochem Biophys Res Commun. 2016;478(3):1338–43.
Doll S, Proneth B, Tyurina YY, Panzilius E, Kobayashi S, Ingold I, et al. ACSL4 dictates ferroptosis sensitivity by shaping cellular lipid composition. Nat Chem Biol. 2017;13(1):91–8.
Hsieh H, Vignesh KS, Deepe GS, Choubey D, Shertzer HG, Genter MB. Mechanistic studies of the toxicity of zinc gluconate in the olfactory neuronal cell line Odora. Toxicol vitro: Int J Published Association BIBRA. 2016;35:24–30.
Article CAS Google Scholar
Wang H, Liu C, Zhao YX, Gao G. Mitochondria regulation in ferroptosis. Eur J Cell Biol. 2020;99(1).
Gao M, Yi J, Zhu J, Minikes AM, Monian P, Thompson CB, et al. Role of Mitochondria in Ferroptosis. Mol Cell. 2019;73(2):354–e633.
Gaschler MM, Hu F, Feng H, Linkermann A, Min W, Stockwell BR. Determination of the Subcellular Localization and Mechanism of Action of Ferrostatins in suppressing ferroptosis. ACS Chem Biol. 2018;13(4):1013–20.
Friedmann Angeli JP, Schneider M, Proneth B, Tyurina YY, Tyurin VA, Hammond VJ, et al. Inactivation of the ferroptosis regulator Gpx4 triggers acute renal failure in mice. Nat Cell Biol. 2014;2014 16(12):12.
Google Scholar
Murphy MP. How mitochondria produce reactive oxygen species. Biochem J. 2009;417(Pt 1):1.
Liu Ye, Lu S, Wu Ll, Yang L, Yang L, Wang J. The diversified role of mitochondria in ferroptosis in cancer. Cell Death Disease 2023. 2023;14(8):8.
Gao M, Monian P, Quadri N, Ramasamy R, Jiang X. Glutaminolysis and transferrin regulate Ferroptosis. Mol Cell. 2015;59(2):298.
Murphy TH, Miyamoto M, Sastre A, Schnaar RL, Coyle JT. Glutamate toxicity in a neuronal cell line involves inhibition of cystine transport leading to oxidative stress. Neuron. 1989;2(6):1547–58.
Shirlee Tan BSP, David Schubert BSP, Pamela Maher BSP, Oxytosis. A novel form of programmed cell death. Curr Top Med Chem. 2001;1(6):497–506.
Article Google Scholar
Hirschhorn T, Stockwell BR. The development of the concept of ferroptosis. Free Radic Biol Med. 2019;133:130–43.
Enke U, Seyfarth L, Schleussner E, Markert UR. Impact of PUFA on early immune and fetal development. Br J Nutr. 2008;100(6):1158–68.
Matsushita M, Freigang S, Schneider C, Conrad M, Bornkamm GW, Kopf M. T cell lipid peroxidation induces ferroptosis and prevents immunity to infection. J Exp Med. 2015;212(4):555–68.
Gujja P, Rosing DR, Tripodi DJ, Shizukuda Y. Iron overload cardiomyopathy: better understanding of an increasing disorder. J Am Coll Cardiol. 2010;56(13):1001–12.
Yuan H, Pratte J, Giardina C. Ferroptosis and its potential as a therapeutic target. Biochem Pharmacol. 2021;186:114486.
Zhang Y, Sun C, Zhao C, Hao J, Zhang Y, Fan B, et al. Ferroptosis inhibitor SRS 16–86 attenuates ferroptosis and promotes functional recovery in contusion spinal cord injury. Brain Res. 2019;1706:48–57.
Magtanong L, Dixon SJ. Ferroptosis and Brain Injury. Dev Neurosci. 2018;40(5–6):382–95.
Cassier-Chauvat C, Marceau F, Farci S, Ouchane S, Chauvat F. The glutathione system: a journey from Cyanobacteria to higher eukaryotes. Antioxid (Basel). 2023;12(6).
Tan M, Yin Y, Ma X, Zhang J, Pan W, Tan M, et al. Glutathione system enhancement for cardiac protection: pharmacological options against oxidative stress and ferroptosis. Cell Death Dis. 2023;14(2):131.
Article PubMed PubMed Central Google Scholar
Bao WD, Pang P, Zhou XT, Hu F, Xiong W, Chen K, et al. Loss of ferroportin induces memory impairment by promoting ferroptosis in Alzheimer’s disease. Cell Death Differ. 2021;28(5):1548–62.
Zhao Y, Liu Y, Xu Y, Li K, Zhou L, Qiao H, et al. The role of ferroptosis in blood–brain barrier Injury. Cell Mol Neurobiol. 2023;43(1):223–36.
Xu Y, Li K, Zhao Y, Zhou L, Liu Y, Zhao J. Role of ferroptosis in stroke. Cell Mol Neurobiol. 2023;43(1):205–22.
Tian X, Li X, Pan M, Yang LZ, Li Y, Fang W. Progress of ferroptosis in ischemic stroke and therapeutic targets. Cell Mol Neurobiol. 2024;44(1):25.
Lei G, Zhuang L, Gan B. Targeting ferroptosis as a vulnerability in cancer. Nat Rev Cancer. 2022;22(7):381–96.
Feng S, Tang D, Wang Y, Li X, Bao H, Tang C, et al. The mechanism of ferroptosis and its related diseases. Mol Biomed. 2023;4(1):33.
Siegel RM. Caspases at the crossroads of immune-cell life and death. Nat Rev Immunol. 2006;6(4):308–17.
Man SM, Karki R, Kanneganti TD. Molecular mechanisms and functions of pyroptosis, inflammatory caspases and inflammasomes in infectious diseases. Immunol Rev. 2017;277(1):61–75.
Brennan MA, Cookson BT. Salmonella induces macrophage death by caspase-1-dependent necrosis. Mol Microbiol. 2000;38(1):31–40.
Zychlinsky A, Prevost MC, Sansonetti PJ. Shigella flexneri induces apoptosis in infected macrophages. Nature. 1992;358(6382):167–9.
Latz E, Xiao TS, Stutz A. Activation and regulation of the inflammasomes. Nat Reviews Immunol 2013. 2013;13(6):6.
Lamkanfi M, Dixit VM. Mechanisms and functions of inflammasomes. Cell. 2014;157(5):1013–22.
Rathinam VAK, Fitzgerald KA. Inflammasome complexes: emerging mechanisms and Effector functions. Cell. 2016;165(4):792–800.
Man SM, Hopkins LJ, Nugent E, Cox S, Glück IM, Tourlomousis P, et al. Inflammasome activation causes dual recruitment of NLRC4 and NLRP3 to the same macromolecular complex. Proc Natl Acad Sci USA. 2014;111(20):7403–8.
Liu S, Du J, Li D, Yang P, Kou Y, Li C, et al. Oxidative stress induced pyroptosis leads to osteogenic dysfunction of MG63 cells. J Mol Histol. 2020;51(3):221–32.
Zhou B, Zhang J, Xs L, Hz C, Yl A, Cheng K, et al. Tom20 senses iron-activated ROS signaling to promote melanoma cell pyroptosis. Cell Res. 2018;28(12):1171–85.
Zhao C, Yu D, He Z, Bao L, Feng L, Chen L, et al. Endoplasmic reticulum stress-mediated autophagy activation is involved in cadmium-induced ferroptosis of renal tubular epithelial cells. Free Radic Biol Med. 2021;175:236–48.
Shen B, Mei M, Ai S, Liao X, Li N, Xiang S et al. TRPC6 inhibits renal tubular epithelial cell pyroptosis through regulating zinc influx and alleviates renal ischemia-reperfusion injury. FASEB Journal: Official Publication Federation Am Soc Experimental Biology. 2022;36(10).
Gu X, Wang Y, He Y, Zhao B, Zhang Q, Li S. MiR-1656 targets GPX4 to trigger pyroptosis in broilers kidney tissues by activating NLRP3 inflammasome under Se deficiency. J Nutr Biochem. 2022;105.
Liao J, Hu Z, Li Q, Li H, Chen W, Huo H, et al. Endoplasmic reticulum stress contributes to Copper-Induced pyroptosis via regulating the IRE1α-XBP1 pathway in Pig Jejunal epithelial cells. J Agric Food Chem. 2022;70(4):1293–303.
Pi S, Nie G, Wei Z, Yang F, Wang C, Xing C, et al. Inhibition of ROS/NLRP3/Caspase-1 mediated pyroptosis alleviates excess molybdenum-induced apoptosis in duck renal tubular epithelial cells. Ecotoxicol Environ Saf. 2021;208:111528.
Yan H, Luo B, Wu X, Guan F, Yu X, Zhao L, et al. Cisplatin induces pyroptosis via activation of MEG3/NLRP3/caspase-1/GSDMD pathway in Triple-negative breast Cancer. Int J Biol Sci. 2021;17(10):2606.
Li Y, Xia W, Wu M, Yin J, Wang Q, Li S, et al. Activation of GSDMD contributes to acute kidney injury induced by cisplatin. Am J Physiol - Ren Physiol. 2020;318(1):F96–106.
Li RY, Zheng ZY, Li ZM, Heng JH, Zheng YQ, Deng DX et al. Cisplatin-induced pyroptosis is mediated via the CAPN1/CAPN2-BAK/BAX-caspase-9-caspase-3-GSDME axis in esophageal cancer. Chemico-Biol Interact. 2022;361.
Zhang Cc L, Cg W, Yf X, Lh H, Xh Z, Qz, et al. Chemotherapeutic paclitaxel and cisplatin differentially induce pyroptosis in A549 lung cancer cells via caspase-3/GSDME activation. Apoptosis. 2019;24(3–4):312–25.
Article PubMed Google Scholar
Yu J, Li S, Qi J, Chen Z, Wu Y, Guo J et al. Cleavage of GSDME by caspase-3 determines lobaplatin-induced pyroptosis in colon cancer cells. Cell Death Dis. 2019;10(3).
Chen J, Ge L, Shi X, Liu J, Ruan H, Heng D, et al. Lobaplatin induces pyroptosis in Cervical Cancer cells via the Caspase-3/GSDME Pathway. Anti-cancer Agents Med Chem. 2022;22(11):2091–7.
Chen ZD, Xu G, Wu D, Wu SH, Gong L, Li ZH et al. Lobaplatin induces pyroptosis through regulating cIAP1/2, Ripoptosome and ROS in nasopharyngeal carcinoma. Biochem Pharmacol. 2020;177.
Tang J, Bei M, Zhu J, Xu G, Chen D, Jin X et al. Acute cadmium exposure induces GSDME-mediated pyroptosis in triple-negative breast cancer cells through ROS generation and NLRP3 inflammasome pathway activation. Environ Toxicol Pharmacol. 2021;87.
Long Y, Liu X, Xz T, Cx J, Sw C. ROS-induced NLRP3 inflammasome priming and activation mediate PCB 118- induced pyroptosis in endothelial cells. Ecotoxicol Environ Saf. 2020;189:109937.
Fang Y, Tian S, Pan Y, Li W, Wang Q, Tang Y, et al. Pyroptosis: a new frontier in cancer. Volume 121. Biomedicine & pharmacotherapy = Biomedecine & pharmacotherapie; 2020.
Murao A, Aziz M, Wang H, Brenner M, Wang P. Release mechanisms of major DAMPs. Apoptosis. 2021;26(3):152.
Zindel J, Kubes P. DAMPs, PAMPs, and LAMPs in immunity and sterile inflammation. Annu Rev Pathol. 2020;15:493–518.
Miao EA, Rajan JV, Aderem A. Caspase-1-induced pyroptotic cell death. Immunol Rev. 2011;243(1):206–14.
Jorgensen I, Rayamajhi M, Miao EA. Programmed cell death as a defence against infection. Nat Reviews Immunol 2017. 2017;17(3):3.
Ranson N, Kunde D, Eri R. Regulation and sensing of inflammasomes and their impact on Intestinal Health. Int J Mol Sci. 2017;18(11).
Nagata S, Tanaka M. Programmed cell death and the immune system. Nat Reviews Immunol 2017. 2017;17(5):5.
An J, Kim SY, Yang EG, Chung HS. A fluorescence-polarization-based lipopolysaccharide-Caspase-4 Interaction Assay for the development of inhibitors. Molecules. 2022;27(8).
Hu L, Chen M, Chen X, Zhao C, Fang Z, Wang H et al. Chemotherapy-induced pyroptosis is mediated by BAK/BAX-caspase-3-GSDME pathway and inhibited by 2-bromopalmitate. Cell Death & Disease 2020 11:4. 2020;11(4):1–17.
Kostura MJ, Tocci MJ, Limjuco G, Chin J, Cameron P, Hillman AG, et al. Identification of a monocyte specific pre-interleukin 1 beta convertase activity. Proc Natl Acad Sci USA. 1989;86(14):5227–31.
Black RA, Kronheim SR, Sleath PR. Activation of interleukin-1 beta by a co-induced protease. FEBS Lett. 1989;247(2):386–90.
Ghayur T, Banerjee S, Hugunin M, Butler D, Herzog L, Carter A, et al. Caspase-1 processes IFN-gamma-inducing factor and regulates LPS-induced IFN-gamma production. Nature. 1997;386(6625):619–23.
Thornberry NA, Bull HG, Calaycay JR, Chapman KT, Howard AD, Kostura MJ, et al. A novel heterodimeric cysteine protease is required for interleukin-1 beta processing in monocytes. Nature. 1992;356(6372):768–74.
Liu X, Zhang Z, Ruan J, Pan Y, Magupalli VG, Wu H, et al. Inflammasome-activated gasdermin D causes pyroptosis by forming membrane pores. Nat 2016. 2016;535(7610):7610.
Aglietti RA, Estevez A, Gupta A, Ramirez MG, Liu PS, Kayagaki N, et al. GsdmD p30 elicited by caspase-11 during pyroptosis forms pores in membranes. Proc Natl Acad Sci USA. 2016;113(28):7858–63.
Chen X, He WT, Hu L, Li J, Fang Y, Wang X, et al. Pyroptosis is driven by non-selective gasdermin-D pore and its morphology is different from MLKL channel-mediated necroptosis. Cell Res 2016. 2016;26(9):9.
Ding J, Wang K, Liu W, She Y, Sun Q, Shi J, et al. Pore-forming activity and structural autoinhibition of the gasdermin family. Nature. 2016;535(7610):111–6.
Kovacs SB, Miao EA, Gasdermins. Effectors of Pyroptosis. Trends Cell Biol. 2017;27(9):673–84.
Lemasters JJ. Molecular mechanisms of cell death. Essent Concepts Mol Pathol. 2020.
Galluzzi L, Vitale I, Aaronson SA, Abrams JM, Adam D, Agostinis P, et al. Molecular mechanisms of cell death: recommendations of the nomenclature Committee on Cell Death 2018. Cell Death Differ 2018. 2018;25(3):3.
Broz P, Dixit VM. Inflammasomes: mechanism of assembly, regulation and signalling. Nat Reviews Immunol 2016. 2016;16(7):7.
Rogers C, Fernandes-Alnemri T, Mayes L, Alnemri D, Cingolani G, Alnemri ES. Cleavage of DFNA5 by caspase-3 during apoptosis mediates progression to secondary necrotic/pyroptotic cell death. Nat Commun. 2017;8.
Wang Y, Yin B, Li D, Wang G, Han X, Sun X. GSDME mediates caspase-3-dependent pyroptosis in gastric cancer. Biochem Biophys Res Commun. 2018;495(1):1418–25.
Jiang M, Qi L, Li L, Li Y. The caspase-3/GSDME signal pathway as a switch between apoptosis and pyroptosis in cancer. Cell Death Discovery 2020. 2020;6(1):1.
Taabazuing CY, Okondo MC, Bachovchin DA. Pyroptosis and apoptosis pathways engage in bidirectional crosstalk in Monocytes and macrophages. Cell Chem Biology. 2017;24(4):507–e144.
Martinon F, Mayor A, Tschopp J. The inflammasomes: guardians of the body. Annu Rev Immunol. 2009;27:229–65.
Harijith A, Ebenezer DL, Natarajan V. Reactive oxygen species at the crossroads of inflammasome and inflammation. Front Physiol. 2014;0:352.
Cridland JA, Curley EZ, Wykes MN, Schroder K, Sweet MJ, Roberts TL et al. The mammalian PYHIN gene family: phylogeny, evolution and expression. BMC Evol Biol. 2012;12(1).
Bosso M, Kirchhoff F. Emerging role of PYHIN Proteins as antiviral restriction factors. Viruses. 2020;12(12).
Sanz AB, Sanchez-Niño MD, Izquierdo MC, Gonzalez-Espinoza L, Ucero AC, Poveda J, et al. Macrophages and recently identified forms of cell death. Int Rev Immunol. 2014;33(1):9–22.
Harijith A, Ebenezer DL, Natarajan V. Reactive oxygen species at the crossroads of inflammasome and inflammation. Front Physiol. 2014;5.
Fernandes-Alnemri T, Wu J, Yu JW, Datta P, Miller B, Jankowski W, et al. The pyroptosome: a supramolecular assembly of ASC dimers mediating inflammatory cell death via caspase-1 activation. Cell Death Differ 2007. 2007;14(9):9.
Alnemri ES. Sensing cytoplasmic danger signals by the inflammasome. J Clin Immunol. 2010;30(4):512–9.
Bergsbaken T, Fink SL, Cookson BT. Pyroptosis: host cell death and inflammation. Nat Rev Microbiol. 2009;7(2):99–109.
Duncan JA, Gao X, Huang MT-H, O’Connor BP, Thomas CE, Willingham SB et al. Neisseria gonorrhoeae activates the proteinase cathepsin B to mediate the signaling activities of the NLRP3 and ASC-containing inflammasome. Journal of immunology (Baltimore, Md: 1950). 2009;182(10):6460-9.
Kayagaki N, Stowe IB, Lee BL, O’Rourke K, Anderson K, Warming S, et al. Caspase-11 cleaves gasdermin D for non-canonical inflammasome signalling. Nature. 2015;526(7575):666–71.
Mitchell PS, Sandstrom A, Vance RE. The NLRP1 inflammasome: new mechanistic insights and unresolved mysteries. Curr Opin Immunol. 2019;60:37–45.
Brydges SD, Broderick L, McGeough MD, Pena CA, Mueller JL, Hoffman HM. Divergence of IL-1, IL-18, and cell death in NLRP3 inflammasomopathies. J Clin Invest. 2013;123(11):4695–705.
Papin S, Duquesnoy P, Cazeneuve C, Pantel J, Coppey-Moisan M, Dargemont C, et al. Alternative splicing at the MEFV locus involved in familial Mediterranean fever regulates translocation of the marenostrin/pyrin protein to the nucleus. Hum Mol Genet. 2000;9(20):3001–9.
Moghaddas F, Llamas R, De Nardo D, Martinez-Banaclocha H, Martinez-Garcia JJ, Mesa-Del-Castillo P, et al. A novel pyrin-Associated Autoinflammation with Neutrophilic Dermatosis mutation further defines 14-3-3 binding of pyrin and distinction to familial Mediterranean Fever. Ann Rheum Dis. 2017;76(12):2085–94.
Demircan C, Akdogan N, Elmas L. Nicolau Syndrome secondary to Subcutaneous Glatiramer acetate injection. Int J Low Extrem Wounds. 2023;22(1):149–51.
Karki R, Sharma BR, Tuladhar S, Williams EP, Zalduondo L, Samir P, et al. Synergism of TNF-α and IFN-γ triggers inflammatory cell death, tissue damage, and Mortality in SARS-CoV-2 infection and cytokine shock syndromes. Cell. 2021;184(1):149–e6817.
Yap JKY, Moriyama M, Iwasaki A. Inflammasomes and Pyroptosis as therapeutic targets for COVID-19. J Immunol. 2020;205(2):307–12.
Ratajczak MZ, Kucia M. SARS-CoV-2 infection and overactivation of Nlrp3 inflammasome as a trigger of cytokine storm and risk factor for damage of hematopoietic stem cells. Leukemia. 2020;34(7):1726–9.
Yan Z, Qi W, Zhan J, Lin Z, Lin J, Xue X, et al. Activating Nrf2 signalling alleviates osteoarthritis development by inhibiting inflammasome activation. J Cell Mol Med. 2020;24(22):13046–57.
Wu Y, Zhang J, Yu S, Li Y, Zhu J, Zhang K, et al. Cell pyroptosis in health and inflammatory diseases. Cell Death Discovery. 2022;8(1):191.
Wei X, Xie F, Zhou X, Wu Y, Yan H, Liu T, et al. Role of pyroptosis in inflammation and cancer. Cell Mol Immunol 2022. 2022;19(9):9.
Chang SC, Yang WV. Hyperglycemia, tumorigenesis, and chronic inflammation. Crit Rev Oncol Hematol. 2016;108:146–53.
Balkwill F, Mantovani A. Inflammation and cancer: back to Virchow? Lancet. 2001;357(9255):539–45.
Cowan AE, Moraru II, Schaff JC, Slepchenko BM, Loew LM. Chapter 8 - spatial modeling of Cell Signaling Networks. In: Asthagiri AR, Arkin AP, editors. Methods in Cell Biology. 2012;110:Academic, 195–221.
Van Cleave C, Crans DC. The first-row transition metals in the periodic table of Medicine. Inorganics. 2019;7(9):111.
Chang CJ. Searching for harmony in transition-metal signaling. Nature Chemical Biology 2015 11:10. 2015;11(10):744-7.
Mendel RR, Smith AG, Marquet A, Warren MJ. Metal and cofactor insertion. Nat Prod Rep. 2007;24(5):963–71.
Parigi G, Ravera E, Luchinat C. Paramagnetic effects in NMR for protein structures and ensembles: studies of metalloproteins. Curr Opin Struct Biol. 2022;74.
Baksh KA, Zamble DB. Allosteric control of metal-responsive transcriptional regulators in bacteria. J Biol Chem. 2020;295(6):1673–84.
Yamasaki S, Sakata-Sogawa K, Hasegawa A, Suzuki T, Kabu K, Sato E, et al. Zinc is a novel intracellular second messenger. J Cell Biol. 2007;177(4):637–45.
Ackerman CM, Lee S, Chang CJ. Analytical Methods for Imaging Metals in Biology: from transition metal metabolism to Transition Metal Signaling. Anal Chem. 2017;89(1):22–41.
Holm RH, Kennepohl P, Solomon EI. Structural and functional aspects of Metal sites in Biology. Chem Rev. 1996;96(7):2239–314.
Chiarelli R, Roccheri MC. Heavy Metals and Metalloids as Autophagy Inducing Agents: Focus on Cadmium and Arsenic. Cells 2012, Vol 1, Pages 597–616. 2012;1(3):597–616.
Crichton R. Iron Metabolism: From Molecular Mechanisms to Clinical Consequences: 3rd Edition. Iron Metabolism: From Molecular Mechanisms to Clinical Consequences: 3rd Edition. 2009:1-461.
Dutt S, Hamza I, Bartnikas TB. Molecular mechanisms of Iron and Heme Metabolism. Annu Rev Nutr. 2022;42:311–35.
Zhang M, Liu Z, Le Y, Gu Z, Zhao H. Iron-Sulfur Clusters: A Key Factor of Regulated Cell Death in Cancer. Oxidative medicine and cellular longevity. 2022;2022.
Hurrell R, Egli I. Iron bioavailability and dietary reference values. Am J Clin Nutr. 2010;91(5).
Bruzzese A, Martino EA, Mendicino F, Lucia E, Olivito V, Bova C et al. Iron chelation therapy. Eur J Haematol. 2023;110(5).
Hatcher HC, Singh RN, Torti FM, Torti SV. Synthetic and natural iron chelators: therapeutic potential and clinical use. Future Med Chem. 2009;1(9):1643–70.
Krzywoszyńska K, Witkowska D, Swiatek-kozlowska J, Szebesczyk A, Kozłowski H. General aspects of metal ions as signaling agents in health and disease. Biomolecules. 2020;10(10):1–30.
Duck KA, Connor JR. Iron uptake and transport across physiological barriers. Biometals. 2016;29(4):573.
West AR, Oates PS. Mechanisms of heme iron absorption: current questions and controversies. World J Gastroenterology: WJG. 2008;14(26):4101.
Article PubMed Central CAS Google Scholar
White C, Yuan X, Schmidt PJ, Bresciani E, Samuel TK, Campagna D, et al. HRG1 is essential for heme transport from the phagolysosome of macrophages during erythrophagocytosis. Cell Metabol. 2013;17(2):261.
Rajagopal A, Rao AU, Amigo J, Tian M, Upadhyay SK, Hall C, et al. Haem homeostasis is regulated by the conserved and concerted functions of HRG-1 proteins. Nature. 2008;453(7198):1127.
Uzel C. MEC. Absorption of heme iron.
Mackenzie B, Garrick MD. Iron imports. II. Iron uptake at the apical membrane in the intestine. Am J Physiol Gastrointest Liver Physiol. 2005;289(6).
Andrews NC. Forging a field: the golden age of iron biology. Blood. 2008;112(2):219.
Zhang N, Yu X, Xie J, Xu H. New insights into the role of Ferritin in Iron Homeostasis and neurodegenerative diseases. Mol Neurobiol. 2021;58(6):2812–23.
Ganz T. Cellular iron: ferroportin is the only way out. Cell Metabol. 2005;1(3):155–7.
Vulpe CD, Kuo YM, Murphy TL, Cowley L, Askwith C, Libina N, et al. Hephaestin, a ceruloplasmin homologue implicated in intestinal iron transport, is defective in the sla mouse. Nat Genet. 1999;21(2):195–9.
Gomme PT, McCann KB. Transferrin: structure, function and potential therapeutic actions. Drug Discovery Today. 2005;10(4):267–73.
Abbaspour N, Hurrell R, Kelishadi R. Review on iron and its importance for human health. J Res Med Sciences: Official J Isfahan Univ Med Sci. 2014;19(2):164.
Haber F, Weiss J, Seph JO, Eiss W. The Catalytic Decom position o f hydrogen peroxide by Iron Salts* by F r it z H aber and.
Barbehenn R, Dodick T, Poopat U, Spencer B. Fenton-type reactions and iron concentrations in the midgut fluids of tree-feeding caterpillars. Arch Insect Biochem Physiol. 2005;60(1):32–43.
Sukhbaatar N, Weichhart T. Iron Regulation: macrophages in control. Pharmaceuticals. 2018;11(4).
Yang WS, Sriramaratnam R, Welsch ME, Shimada K, Skouta R, Viswanathan VS, et al. Regulation of ferroptotic cancer cell death by GPX4. Cell. 2014;156(1–2):317–31.
Lei P, Bai T, Sun Y. Mechanisms of ferroptosis and relations with regulated cell death: a review. Front Physiol. 2019;10(FEB):139.
Bouchaoui H, Mahoney-Sanchez L, Garçon G, Berdeaux O, Alleman LY, Devos D, et al. ACSL4 and the lipoxygenases 15/15B are pivotal for ferroptosis induced by iron and PUFA dyshomeostasis in dopaminergic neurons. Free Radic Biol Med. 2023;195:145–57.
Hider R, Aviles MV, Chen YL, Latunde-Dada GO. The role of GSH in Intracellular Iron trafficking. Int J Mol Sci. 2021;22(3):1–13.
Hambright WS, Fonseca RS, Chen L, Na R, Ran Q. Ablation of ferroptosis regulator glutathione peroxidase 4 in forebrain neurons promotes cognitive impairment and neurodegeneration. Redox Biol. 2017;12:8–17.
Ayton S, Faux NG, Bush AI, Weiner MW, Aisen P, Petersen R et al. Ferritin levels in the cerebrospinal fluid predict Alzheimer’s disease outcomes and are regulated by APOE. Nat Commun. 2015;6.
Ashraf A, Jeandriens J, Parkes HG, So PW. Iron dyshomeostasis, lipid peroxidation and perturbed expression of cystine/glutamate antiporter in Alzheimer’s disease: Evidence of ferroptosis. Redox Biol. 2020;32.
Wu A, Feng B, Yu J, Yan L, Che L, Zhuo Y et al. Fibroblast growth factor 21 attenuates iron overload-induced liver injury and fibrosis by inhibiting ferroptosis. Redox Biol. 2021;46.
Adedoyin O, Boddu R, Traylor A, Lever JM, Bolisetty S, George JF, et al. Heme oxygenase-1 mitigates ferroptosis in renal proximal tubule cells. Am J Physiol Ren Physiol. 2018;314(5):F702–14.
Menon AV, Liu J, Tsai HP, Zeng L, Yang S, Asnani A, et al. Excess heme upregulates heme oxygenase 1 and promotes cardiac ferroptosis in mice with sickle cell disease. Blood. 2022;139(6):936–41.
Ma C, Wu X, Zhang X, Liu X, Deng G. Heme oxygenase-1 modulates ferroptosis by fine-tuning levels of intracellular iron and reactive oxygen species of macrophages in response to Bacillus Calmette-Guerin infection. Front Cell Infect Microbiol. 2022;12.
Zhang P, Chen L, Zhao Q, Du X, Bi M, Li Y, et al. Ferroptosis was more initial in cell death caused by iron overload and its underlying mechanism in Parkinson’s disease. Free Radic Biol Med. 2020;152:227–34.
Liu Y, Gu W. p53 in ferroptosis regulation: the new weapon for the old guardian. Cell Death Differ. 2022;29(5):895–910.
Mancias JD, Wang X, Gygi SP, Harper JW, Kimmelman AC. Quantitative proteomics identifies NCOA4 as the cargo receptor mediating ferritinophagy. Nat 2014. 2014;509(7498):7498.
Kaur J, Debnath J. Autophagy at the crossroads of catabolism and anabolism. Nat Reviews Mol Cell Biology 2015. 2015;16(8):8.
Gao M, Monian P, Pan Q, Zhang W, Xiang J, Jiang X. Ferroptosis is an autophagic cell death process. Cell Res. 2016;26(9):1021.
Gryzik M, Asperti M, Denardo A, Arosio P, Poli M. NCOA4-mediated ferritinophagy promotes ferroptosis induced by erastin, but not by RSL3 in HeLa cells. Biochim et Biophys acta Mol cell Res. 2021;1868(2).
Hou W, Xie Y, Song X, Sun X, Lotze MT, Zeh HJ, et al. Autophagy promotes ferroptosis by degradation of ferritin. Autophagy. 2016;12(8):1425–8.
Bellelli R, Federico G, Matte A, Colecchia D, Iolascon A, Chiariello M, et al. NCOA4 Deficiency impairs systemic Iron homeostasis. Cell Rep. 2016;14(3):411–21.
Sun K, Li C, Liao S, Yao X, Ouyang Y, Liu Y, et al. Ferritinophagy, a form of autophagic ferroptosis: new insights into cancer treatment. Front Pharmacol. 2022;13:4548.
Tang YS, Zhao YH, Zhong Y, Li XZ, Pu JX, Luo YC, et al. Neferine inhibits LPS-ATP-induced endothelial cell pyroptosis via regulation of ROS/NLRP3/Caspase-1 signaling pathway. Inflamm Research: Official J Eur Histamine Res Soc [et al]. 2019;68(9):727–38.
Jomova K, Makova M, Alomar SY, Alwasel SH, Nepovimova E, Kuca K et al. Essential metals in health and disease. Chemico-Biol Interact. 2022;367.
Hübner C, Haase H. Interactions of zinc- and redox-signaling pathways. Redox Biol. 2021;41.
Kimura T, Kambe T. The functions of Metallothionein and ZIP and ZnT transporters: an overview and perspective. Int J Mol Sci. 2016;17(3).
Bin BH, Seo J, Kim ST, Function. Structure, and Transport Aspects of ZIP and ZnT Zinc Transporters in Immune Cells. Journal of Immunology Research. 2018;2018.
Maret W. The redox biology of redox-inert zinc ions. Free Radic Biol Med. 2019;134:311–26.
Kręzel A, Maret W. Dual nanomolar and picomolar zn(II) binding properties of metallothionein. J Am Chem Soc. 2007;129(35):10911–21.
Krężel A, Maret W. The functions of metamorphic metallothioneins in zinc and copper metabolism. Int J Mol Sci. 2017;18(6).
Thornalley PJ, Vašák M. Possible role for metallothionein in protection against radiation-induced oxidative stress. Kinetics and mechanism of its reaction with superoxide and hydroxyl radicals. Biochim Biophys Acta. 1985;827(1):36–44.
Tapiero H, Tew KD. Trace elements in human physiology and pathology: zinc and metallothioneins. Biomed Pharmacotherapy. 2003;57(9):399–411.
Maret W. Zinc coordination environments in proteins as redox sensors and signal transducers. Antioxid Redox Signal. 2006;8(9–10):1419–41.
Jacob C, Maret W, Vallee BL. Control of zinc transfer between thionein, metallothionein, and zinc proteins. Proc Natl Acad Sci USA. 1998;95(7):3489–94.
Jiang LJ, Maret W, Vallee BL. The glutathione redox couple modulates zinc transfer from metallothionein to zinc-depleted sorbitol dehydrogenase. Proc Natl Acad Sci USA. 1998;95(7):3483–8.
Maret W, Vallee BL. Thiolate ligands in metallothionein confer redox activity on zinc clusters. Proc Natl Acad Sci USA. 1998;95(7):3478–82.
Samman S. Dietary versus cellular zinc: the antioxidant paradox. Free Radic Biol Med. 1993;14(1):95–6.
Schrauzer GN. Anticarcinogenic effects of selenium. Cell Mol Life Sci. 2000;57(13–14):1864–73.
Schrauzer GN. Antioxidant supplementation increases skin cancer risk, or, why zinc should not be considered an antioxidant. J Nutr. 2008;138(4):820.
Chen PH, Wu J, Xu Y, Ding CKC, Mestre AA, Lin CC et al. Zinc transporter ZIP7 is a novel determinant of ferroptosis. Cell Death Dis. 2021;12(2).
Ge Mh, Tian H, Mao L, Li D, Jq L, Hs H, et al. Zinc attenuates ferroptosis and promotes functional recovery in contusion spinal cord injury by activating Nrf2/GPX4 defense pathway. CNS Neurosci Ther. 2021;27(9):1023–40.
Ferrer X, Moreno JJ. Effects of copper, iron and zinc on oedema formation induced by phospholipase A2. Comparative biochemistry and physiology C. Comp Pharmacol Toxicol. 1992;102(2):325–7.
CAS Google Scholar
Zhang Y, Aizenman E, DeFranco DB, Rosenberg PA. Intracellular zinc release, 12-lipoxygenase activation and MAPK dependent neuronal and oligodendroglial death. Mol Med. 2007;13(7–8):350–5.
Morita Y, Sawada M, Seno H, Takaishi S, Fukuzawa H, Miyake N, et al. Identification of xanthine dehydrogenase/xanthine oxidase as a rat paneth cell zinc-binding protein. Biochimica et Biophysica Acta (BBA) -. Mol Cell Res. 2001;1540(1):43–9.
Wang Z, Li X, Du S, Sun X, Huang J, Shao Y. Protective effects of Zinc on Salmonella Invasion, intestinal morphology and Immune Response of Young pigeons infected with Salmonella enterica Serovar Typhimurium. Biol Trace Elem Res. 2022;200(11):4817–27.
Zhao Z, Hu X, Wang J, Wang J, Hou Y, Chen S. Zinc finger E-Box binding homeobox 2 (ZEB2)-induced astrogliosis protected neuron from pyroptosis in cerebral ischemia and reperfusion injury. Bioengineered. 2021;12(2):12917–30.
Mehdi Y, Hornick JL, Istasse L, Dufrasne I. Selenium in the environment, metabolism and involvement in body functions. Molecules. 2013;18(3):3292–311.
Ingold I, Berndt C, Schmitt S, Doll S, Poschmann G, Buday K, et al. Selenium utilization by GPX4 is required to Prevent Hydroperoxide-Induced ferroptosis. Cell. 2018;172(3):409–e2221.
Yin K, Sun X, Zheng Y, Zhang W, Lin H. Bisphenol A exacerbates selenium deficiency-induced pyroptosis via the NF-κB/NLRP3/Caspase-1 pathway in chicken trachea. Comp Biochem Physiol C: Toxicol Pharmacol. 2023;263:109488.
PubMed CAS Google Scholar
Conrad M, Proneth B. Selenium: tracing another essential element of ferroptotic cell death. Cell Chem Biology. 2020;27(4):409–19.
Hatfield DL, Gladyshev VN. How selenium has altered our understanding of the genetic code. Mol Cell Biol. 2002;22(11):3565–76.
Stoytcheva ZR, Berry MJ. Transcriptional regulation of mammalian selenoprotein expression. Biochim Biophys Acta. 2009;1790(11):1429–40.
Alim I, Caulfield JT, Chen Y, Swarup V, Geschwind DH, Ivanova E, et al. Selenium drives a Transcriptional Adaptive Program to Block Ferroptosis and treat stroke. Cell. 2019;177(5):1262–e7925.
Yao Y, Chen Z, Zhang H, Chen C, Zeng M, Yunis J, et al. Selenium-GPX4 axis protects follicular helper T cells from ferroptosis. Nat Immunol. 2021;22(9):1127–39.
Fradejas N, Carlson BA, Rijntjes E, Becker NP, Tobe R, Schweizer U. Mammalian Trit1 is a tRNA([Ser]Sec)-isopentenyl transferase required for full selenoprotein expression. Biochem J. 2013;450(2):427–32.
Zhang X, Guo Y, Li H, Han L. FIN56, a novel ferroptosis inducer, triggers lysosomal membrane permeabilization in a TFEB-dependent manner in glioblastoma. J Cancer. 2021;12(22):6610.
Ryu H, Lee J, Zaman K, Kubilis J, Ferrante RJ, Ross BD, et al. Sp1 and Sp3 are oxidative Stress-Inducible, Antideath Transcription Factors in cortical neurons. J Neurosci. 2003;23(9):3597.
Müller A, Cadenas E, Graf P, Sies H. A novel biologically active seleno-organic compound–I. glutathione peroxidase-like activity in vitro and antioxidant capacity of PZ 51 (Ebselen). Biochem Pharmacol. 1984;33(20):3235–9.
Wu H, Luan Y, Wang H, Zhang P, Liu S, Wang P, et al. Selenium inhibits ferroptosis and ameliorates autistic-like behaviors of BTBR mice by regulating the Nrf2/GPx4 pathway. Brain Res Bull. 2022;183:38–48.
Tuo QZ, Masaldan S, Southon A, Mawal C, Ayton S, Bush AI, et al. Characterization of Selenium compounds for anti-ferroptotic activity in neuronal cells and after cerebral ischemia-reperfusion Injury. Neurotherapeutics: J Am Soc Experimental Neurother. 2021;18(4):2682–91.
Wang L, Yang F, Hu M, Chen G, Wang Y, Xu H et al. GPX4 utilization by selenium is required to alleviate cadmium-induced ferroptosis and pyroptosis in sheep kidney. Environ Toxicol. 2023.
Feng J, Yang F, Wu H, Xing C, Xue H, Zhang L, et al. Selenium protects against cadmium-induced cardiac injury by attenuating programmed cell death via PI3K/AKT/PTEN signaling. Environ Toxicol. 2022;37(5):1185–97.
Liu S, Chen Q, Yan L, Ren Y, Fan J, Zhang X et al. Phytosomal tripterine with selenium modification attenuates the cytotoxicity and restrains the inflammatory evolution via inhibiting NLRP3 inflammasome activation and pyroptosis. Int Immunopharmacol. 2022;108.
Mahan DC, Cline TR, Richert B. Effects of dietary levels of selenium-enriched yeast and sodium selenite as selenium sources fed to growing-finishing pigs on performance, tissue selenium, serum glutathione peroxidase activity, carcass characteristics, and loin quality. J Anim Sci. 1999;77(8):2172–9.
Pengcheng X, Xu S, Wei C, Xiaodan H. Yeast selenium exerts an antioxidant effect by regulating the Level of Selenoprotein to Antagonize Cd-Induced pyroptosis of Chicken Liver. Biol Trace Elem Res. 2022;200(9):4079–88.
Song L, Jiang Z, Zhang X, Song Y, Xing Y, Wang G. Selenium Deficiency via the ROS/NLRP3/IL-1β signaling pathway leads to Pyroptosis Injury in Pig Spleen. Biological Trace Element Research; 2023.
Liu Q, Du P, Zhu Y, Zhang X, Cai J, Zhang Z. Thioredoxin reductase 3 suppression promotes colitis and carcinogenesis via activating pyroptosis and necrosis. Cell Mol Life Sci. 2022;79(2).
Aliaga ME, López-Alarcón C, Bridi R, Speisky H. Redox-implications associated with the formation of complexes between copper ions and reduced or oxidized glutathione. J Inorg Biochem. 2016;154:78–88.
Kozlowski H, Kolkowska P, Watly J, Krzywoszynska K, Potocki S. General aspects of metal toxicity. Curr Med Chem. 2014;21(33):3721–40.
Maryon EB, Molloy SA, Ivy K, Yu H, Kaplan JH. Rate and regulation of copper transport by human copper transporter 1 (hCTR1). J Biol Chem. 2013;288(25):18035–46.
Scheiber IF, Mercer JFB, Dringen R. Copper accumulation by cultured astrocytes. Neurochem Int. 2010;56(3):451–60.
Chen L, Min J, Wang F. Copper homeostasis and cuproptosis in health and disease. Signal Transduct Target Therapy. 2022;7(1).
Luza SC, Speisky HC. Liver copper storage and transport during development: implications for cytotoxicity. Am J Clin Nutr. 1996;63(5).
Arredondo M, Núñez MT. Iron and copper metabolism. Mol Aspects Med. 2005;26(4–5):313–27.
Jeong SY, David S. Glycosylphosphatidylinositol-anchored ceruloplasmin is required for iron efflux from cells in the central nervous system. J Biol Chem. 2003;278(29):27144–8.
Bento I, Peixoto C, Zaitsev VN, Lindley PF. Ceruloplasmin revisited: structural and functional roles of various metal cation-binding sites. Acta Crystallogr Sect D Biol Crystallogr. 2007;63(Pt 2):240–8.
Xue Q, Yan D, Chen X, Li X, Kang R, Klionsky DJ et al. Copper-dependent autophagic degradation of GPX4 drives ferroptosis. Autophagy. 2023.
Li F, Wu X, Liu H, Liu M, Yue Z, Wu Z et al. Copper depletion strongly enhances ferroptosis via mitochondrial perturbation and reduction in antioxidative mechanisms. Antioxid (Basel Switzerland). 2022;11(11).
Jhelum P, Santos-Nogueira E, Teo W, Haumont A, Lenoël I, Stys PK, et al. Ferroptosis mediates Cuprizone-Induced loss of oligodendrocytes and demyelination. J Neuroscience: Official J Soc Neurosci. 2020;40(48):9327–41.
Tsvetkov P, Coy S, Petrova B, Dreishpoon M, Verma A, Abdusamad M, et al. Copper induces cell death by targeting lipoylated TCA cycle proteins. Sci (New York NY). 2022;375(6586):1254–61.
Xu Y, Yang W, Han Y, Bian K, Zeng W, Hao L et al. Biomimetic Molybdenum Sulfide-Catalyzed Tumor Ferroptosis and Bioimaging. Small (Weinheim an der Bergstrasse, Germany). 2023:2207544.
Novotny JA, Peterson CA. Molybdenum. Advances in nutrition (Bethesda. Md). 2018;9(3):272–3.
Gu X, Ali T, Chen R, Hu G, Zhuang Y, Luo J et al. In Vivo Studies of Molybdenum-Induced Apoptosis in Kidney Cells of Caprine. Biological Trace Element Research 2015 165:1. 2015;165(1):51 – 8.
Burguera JL, Burguera M. Molybdenum in human whole blood of adult residents of the Merida State (Venezuela). J Trace Elem Med Biol. 2007;21(3):178–83.
Hu Z, Nie G, Luo J, Hu R, Li G, Hu G, et al. Molybdenum and cadmium co-induce pyroptosis via inhibiting Nrf2-Mediated antioxidant defense response in the brain of ducks. Biol Trace Elem Res. 2023;201(2):874–87.
Cao P, Nie G, Luo J, Hu R, Li G, Hu G, et al. Cadmium and molybdenum co-induce pyroptosis and apoptosis via the PTEN/PI3K/AKT axis in the livers of Shaoxing ducks (Anas platyrhynchos). Food Function. 2022;13(4):2142–54.
Zhuang J, Nie G, Hu R, Wang C, Xing C, Li G et al. Inhibition of autophagy aggravates molybdenum-induced mitochondrial dysfunction by aggravating oxidative stress in duck renal tubular epithelial cells. Ecotoxicol Environ Saf. 2021;209.
Leyssens L, Vinck B, Van Der Straeten C, Wuyts F, Maes L. Cobalt toxicity in humans-A review of the potential sources and systemic health effects. Toxicology. 2017;387:43–56.
Liu Y, Zhu W, Ni D, Zhou Z, Gu JH, Zhang W et al. Alpha lipoic acid antagonizes cytotoxicity of cobalt nanoparticles by inhibiting ferroptosis-like cell death. J Nanobiotechnol. 2020;18(1).
Leonard S, Gannett PM, Rojanasakul Y, Schwegler-Berry D, Castranova V, Vallyathan V, et al. Cobalt-mediated generation of reactive oxygen species and its possible mechanism. J Inorg Biochem. 1998;70(3–4):239–44.
Xue S, Xu Y, Xu S, Zhong Y, Ruan G, Ma J, et al. Mitophagy impairment mediates the pathogenesis of CoCrMo particle-induced osteolysis via NLRP3/caspase-1/GSDMD-dependent pyroptosis in macrophages. Chem Eng J. 2022;435:135115.
Liu W, Gan Y, Ding Y, Zhang L, Jiao X, Liu L et al. Autophagy promotes GSDME-mediated pyroptosis via intrinsic and extrinsic apoptotic pathways in cobalt chloride-induced hypoxia reoxygenation-acute kidney injury. Ecotoxicol Environ Saf. 2022;242.
Begum W, Rai S, Banerjee S, Bhattacharjee S, Mondal MH, Bhattarai A, et al. A comprehensive review on the sources, essentiality and toxicological profile of nickel. RSC Adv. 2022;12(15):9139–53.
Zambelli B, Ciurli S. Nickel and human health. Metal Ions Life Sci. 2013;13:321–57.
Barceloux DG, Nickel. J Toxicol Clin Toxicol. 1999;37(2):239–58.
Wei L, Zuo Z, Yang Z, Yin H, Yang Y, Fang J et al. Mitochondria damage and ferroptosis involved in Ni-induced hepatotoxicity in mice. Toxicology. 2022;466.
Chen P, Wang RR, Ma XJ, Liu Q, Ni JZ. Different forms of selenoprotein M differentially affect Aβ aggregation and ROS generation. Int J Mol Sci 2013. 2013;14(3):4385–99.
Ma W, Liu Y, Xu L, Gai X, Sun Y, Qiao S, et al. The role of selenoprotein M in nickel-induced pyroptosis in mice spleen tissue via oxidative stress. Environ Sci Pollut Res Int. 2023;30(12):34270–81.
Douple EB, Richmond RC. A review of platinum complex biochemistry suggests a rationale for combined platinum-radiotherapy. Int J Radiation Oncology*Biology*Physics. 1979;5(8):1335–9.
Rinkovec J. Platinum, palladium, and rhodium in airborne particulate matter. Arh Hig Rada Toksikol. 2019;70(4):224–31.
Guo J, Xu B, Han Q, Zhou H, Xia Y, Gong C, et al. Ferroptosis: a Novel Anti-tumor Action for Cisplatin. Cancer Res Treatment: Official J Korean Cancer Association. 2018;50(2):445.
Ikeda Y, Hamano H, Horinouchi Y, Miyamoto L, Hirayama T, Nagasawa H, et al. Role of ferroptosis in cisplatin-induced acute nephrotoxicity in mice. J Trace Elem Med Biol. 2021;67:126798.
Li Y, Li K, Zhao W, Wang H, Xue X, Chen X et al. VPA improves ferroptosis in tubular epithelial cells after cisplatin-induced acute kidney injury. Front Pharmacol. 2023;14.
Li L, Qiu C, Hou M, Wang X, Huang C, Zou J, et al. Ferroptosis in ovarian cancer: a novel therapeutic strategy. Front Oncol. 2021;11:665945.
Hu Z, Zhang H, Yi B, Yang S, Liu J, Hu J et al. VDR activation attenuate cisplatin induced AKI by inhibiting ferroptosis. Cell Death & Disease 2020 11:1. 2020;11(1):1–11.
Fuertes M, Castilla J, Alonso C, Pérez J. Cisplatin biochemical mechanism of action: from cytotoxicity to induction of cell death through interconnections between apoptotic and necrotic pathways. Curr Med Chem. 2003;10(3):257–66.
Li L, Shang J, Zhang Y, Liu S, Peng Y, Zhou Z, et al. MEG3 is a prognostic factor for CRC and promotes chemosensitivity by enhancing oxaliplatin-induced cell apoptosis. Oncol Rep. 2017;38(3):1383–92.
Wang G, Wang J-J, Zhi-Min Z, Xu X-N, Shi F, Fu X-L. Targeting critical pathways in ferroptosis and enhancing antitumor therapy of platinum drugs for colorectal cancer. Sci Prog. 2023;106(1):00368504221147173.
Yang C, Zhang Y, Lin S, Liu Y, Li W. Suppressing the KIF20A/NUAK1/Nrf2/GPX4 signaling pathway induces ferroptosis and enhances the sensitivity of colorectal cancer to oxaliplatin. Aging. 2021;13(10):13515–34.
Liu Z, Cai J, Jiang G, Wang M, Wu C, Su K, et al. Novel platinum(IV) complexes intervene oxaliplatin resistance in colon cancer via inducing ferroptosis and apoptosis. Eur J Med Chem. 2024;263:115968.
Qi D, Xing L, Shen L, Sun W, Cai C, Xue C, et al. A GSH-depleted platinum(IV) prodrug triggers ferroptotic cell death in breast cancer. Chin Chem Lett. 2022;33(10):4595–9.
Huang ZX, Zhang QY, Wang Y, Chen R, Wang YQ, Huang ZS et al. Inhibition of caspase-3-mediated GSDME-derived pyroptosis aids in noncancerous tissue protection of squamous cell carcinoma patients during cisplatin-based chemotherapy. Am J CANCER Res. 2020;10(12).
Ling YY, Xia XY, Hao L, Wang WJ, Zhang H, Liu LY, et al. Simultaneous photoactivation of cGAS-STING pathway and pyroptosis by platinum (II) triphenylamine complexes for Cancer Immunotherapy. Angewandte Chemie - Int Ed. 2022;61:43.
Rahimzadeh MR, Rahimzadeh MR, Kazemi S, Moghadamnia AA. Cadmium toxicity and treatment: an update. Caspian J Intern Med. 2017;8(3):135.
Genchi G, Sinicropi MS, Lauria G, Carocci A, Catalano A. The effects of Cadmium Toxicity. Int J Environ Res Public Health. 2020;17(11).
Zhang Y, Guo S, Wang S, Li X, Hou D, Li H et al. LncRNA OIP5-AS1 inhibits ferroptosis in prostate cancer with long-term cadmium exposure through miR-128-3p/SLC7A11 signaling. Ecotoxicol Environ Saf. 2021;220.
Marisa D, Aflanie I, Muthmainah N, Suhartono E. Interaction of cadmium-cysteine binding and oxidation of protein causes of blood thrombosis. Journal of Physics: Conference Series. 2019;1374(1):012034-.
Zeng L, Zhou J, Wang X, Zhang Y, Wang M, Su P. Cadmium attenuates testosterone synthesis by promoting ferroptosis and blocking autophagosome-lysosome fusion. Free Radic Biol Med. 2021;176:176–88.
He Z, Shen P, Feng L, Hao H, He Y, Fan G et al. Cadmium induces liver dysfunction and ferroptosis through the endoplasmic stress-ferritinophagy axis. Ecotoxicol Environ Saf. 2022;245.
Wang YJ, Yan J, Yin F, Li L, Qin YG, Meng CY, et al. Role of autophagy in cadmium-induced testicular injury. Hum Exp Toxicol. 2017;36(10):1039–48.
Wu X, Guo X, Wang H, Zhou S, Li L, Chen X et al. A brief exposure to cadmium impairs Leydig cell regeneration in the adult rat testis. Sci Rep. 2017;7(1).
Cupertino MC, Novaes RD, Santos EC, Neves AC, Silva E, Oliveira JA et al. Differential Susceptibility of Germ and Leydig Cells to Cadmium-Mediated Toxicity: Impact on Testis Structure, Adiponectin Levels, and Steroidogenesis. Oxidative medicine and cellular longevity. 2017;2017.
Ji YL, Wang H, Meng C, Zhao XF, Zhang C, Zhang Y, et al. Melatonin alleviates cadmium-induced cellular stress and germ cell apoptosis in testes. J Pineal Res. 2012;52(1):71–9.
Mathur PP, D’Cruz SC. The effect of environmental contaminants on testicular function. Asian J Androl. 2011;13(4):585.
Hu Y, Wu H, Lu C, Xu H, Li B, Guan W et al. Cadmium chloride exposure impairs the growth and behavior of Drosophila via ferroptosis. Sci Total Environ. 2023;865.
Chen PY, Ho YR, Wu MJ, Huang SP, Chen PK, Tai MH et al. Cytoprotective effects of fisetin against hypoxia-induced cell death in PC12 cells.6(1):287–96.
Hong H, Lin X, Xu Y, Tong T, Zhang J, He H et al. Cadmium induces ferroptosis mediated inflammation by activating Gpx4/Ager/p65 axis in pancreatic β-cells. Sci Total Environ. 2022;849.
Darwish WS, Chen Z, Li Y, Wu Y, Chiba H, Hui SP. Identification of cadmium-produced lipid hydroperoxides, transcriptomic changes in antioxidant enzymes, xenobiotic transporters, and pro-inflammatory markers in human breast cancer cells (MCF7) and protection with fat-soluble vitamins. Environ Sci Pollut Res. 2020;27(2):1978–90.
Wu H, Reizel T, Wang YJ, Lapiro JL, Kren BT, Schug J, et al. A negative reciprocal regulatory axis between cyclin D1 and HNF4α modulates cell cycle progression and metabolism in the liver. Proc Natl Acad Sci USA. 2020;117(29):17177–86.
Zhang CY, Lin TJ, Nie GH, Hu RM, Pi SX, Wei ZJ et al. Cadmium and molybdenum co-induce pyroptosis via ROS/PTEN/PI3K/AKT axis in duck renal tubular epithelial cells. Environ Pollut. 2021;272.
Chou X, Ding F, Zhang X, Ding X, Gao H, Wu Q. Sirtuin-1 ameliorates cadmium-induced endoplasmic reticulum stress and pyroptosis through XBP-1s deacetylation in human renal tubular epithelial cells. Arch Toxicol. 2019;93(4):965–86.
Bernhoft RA. Mercury toxicity and treatment: a review of the literature. Journal of environmental and public health. 2012;2012.
Chen J, Ma M, Wang R, Gao M, Hu L, Liu S et al. Roles of glutathione peroxidase 4 on the mercury-triggered ferroptosis in renal cells: implications for the antagonism between selenium and mercury. Metallomics: Integr Biometal Sci. 2023;15(3).
Ahn H, Kim J, Kang SG, Yoon Si, Ko HJ, Kim PH et al. Mercury and arsenic attenuate canonical and non-canonical NLRP3 inflammasome activation. Sci Rep. 2018;8(1).
Gidlow DA. Lead toxicity. Occupational medicine (Oxford, England). 2015;65(5):348 – 56.
Shi F, Yang H, Sun G, Cui J, Li Z, Wang W, et al. Pb induces ferroptosis in choroid plexus epithelial cells via Fe metabolism. Neurotoxicology. 2023;95:107–16.
Su P, Wang D, Cao Z, Chen J, Zhang J. The role of NLRP3 in lead-induced neuroinflammation and possible underlying mechanism. Environ Pollut. 2021;287:117520.
Bolt HM. The Janus face of uranium in toxicology. Arch Toxicol. 2022;96(3):689–90.
Chen X, Wu G, Dang YX, Li Q, Xie MT, Li W, et al. Uranium triggers ferroptosis-like cell death in Vicia faba roots by increasing iron accumulation and inhibiting glutathione peroxidase activity. Environ Exp Bot. 2023;205:105122.
Yin J, Hu N, Yi L, Zhao W, Cheng X, Li G, et al. Identification of ferroptosis biomarker in AHH-1 lymphocytes Associated with Low Dose Radiation. Health Phys. 2021;120(5):541–51.
Claro S, Oshiro MEM, Mortara RA, Paredes-Gamero EJ, Pereira GJS, Smaili SS, et al. γ-Rays-generated ROS induce apoptosis via mitochondrial and cell cycle alteration in smooth muscle cells. Int J Radiat Biol. 2014;90(10):914–27.
Hao R, Ge J, Song X, Li F, Sun-Waterhouse D, Li D. Cadmium induces ferroptosis and apoptosis by modulating miR-34a-5p/Sirt1axis in PC12 cells. Environ Toxicol. 2022;37(1):41–51.
Sun X, Ou Z, Xie M, Kang R, Fan Y, Niu X, et al. HSPB1 as a novel regulator of ferroptotic cancer cell death. Oncogene. 2015;34(45):5617–25.
Download references
This work was supported by the Internal Grant Agency of Faculty of Medicine of Masaryk University (MUNI/A/1587/2023 and MUNI/A/1547/2023).
Author information
Authors and affiliations.
Department of Physiology, Faculty of Medicine, Masaryk University, Kamenice 5, Brno, CZ-625 00, Czech Republic
Michal Masarik & Monika Kratochvilova
Department of Pathological Physiology, Faculty of Medicine, Masaryk University, Kamenice 5, Brno, CZ-625 00, Czech Republic
Frantisek Vana, Zoltan Szabo & Michal Masarik
First Faculty of Medicine, BIOCEV, Charles University, Prumyslova 595, Vestec, CZ-252 50, Czech Republic
Michal Masarik
Regional Centre for Applied Molecular Oncology, Masaryk Memorial Cancer Institute, Zluty kopec 7, Brno, 656 53, Czech Republic
Zoltan Szabo
You can also search for this author in PubMed Google Scholar
Contributions
FV and ZS wrote the manuscript, MK conceived the structure and revised the manuscript, MM revised the manuscript. All authors read and approved the final manuscript.
Corresponding author
Correspondence to Monika Kratochvilova .
Ethics declarations
Ethics approval and consent to participate.
Not applicable.
Competing interests
The authors declare no competing interests.
Additional information
Publisher’s note.
Springer Nature remains neutral with regard to jurisdictional claims in published maps and institutional affiliations.
Rights and permissions
Open Access This article is licensed under a Creative Commons Attribution 4.0 International License, which permits use, sharing, adaptation, distribution and reproduction in any medium or format, as long as you give appropriate credit to the original author(s) and the source, provide a link to the Creative Commons licence, and indicate if changes were made. The images or other third party material in this article are included in the article’s Creative Commons licence, unless indicated otherwise in a credit line to the material. If material is not included in the article’s Creative Commons licence and your intended use is not permitted by statutory regulation or exceeds the permitted use, you will need to obtain permission directly from the copyright holder. To view a copy of this licence, visit http://creativecommons.org/licenses/by/4.0/ . The Creative Commons Public Domain Dedication waiver ( http://creativecommons.org/publicdomain/zero/1.0/ ) applies to the data made available in this article, unless otherwise stated in a credit line to the data.
Reprints and permissions
About this article
Cite this article.
Vana, F., Szabo, Z., Masarik, M. et al. The interplay of transition metals in ferroptosis and pyroptosis. Cell Div 19 , 24 (2024). https://doi.org/10.1186/s13008-024-00127-9
Download citation
Received : 26 March 2024
Accepted : 08 July 2024
Published : 03 August 2024
DOI : https://doi.org/10.1186/s13008-024-00127-9
Share this article
Anyone you share the following link with will be able to read this content:
Sorry, a shareable link is not currently available for this article.
Provided by the Springer Nature SharedIt content-sharing initiative
- Transition metal
- Cardiovascular disease
- Neurological disease
Cell Division
ISSN: 1747-1028
- Submission enquiries: [email protected]

IMAGES
VIDEO
COMMENTS
NUAK family kinase 2 (NUAK2) has been identified as an important mediator for tumor progression in multiple malignancies. Nevertheless, its role in lung adenocarcinoma (LUAD) remains unclear. Jun Fan, Lei Xue, Haoran Lin and Jinhua Luo. Cell Division 2024 19 :23. Research Published on: 28 July 2024.
Cell division is the process by which a parental cell gives rise to two daughter cells. ... Research Highlights 25 Mar 2024 Nature Reviews ... Ahna Skop and Karen Schindler describe a paper that ...
Introduction to cell division. Cell division, or mitosis, is the process by which a mother cell divides its nuclear and cytoplasmic components into two daughter cells. Mitosis is divided into four major phases: prophase, metaphase, anaphase, and telophase. Careful regulation of the cell division program is crucial for proper cell growth ...
Cell division is a highly regulated and carefully orchestrated process. Understanding the mechanisms that promote proper cell division is an important step toward unraveling important questions in cell biology and human health. Early studies seeking to dissect the mechanisms of cell division used classical genetics approaches to identify genes involved in mitosis and deployed biochemical ...
Membrane dynamics respond to changes in cell volume and surface topology. During mitosis, cells alter their volume and surface topology rapidly 50 (Fig. 1 ). With little time for de novo membrane ...
Introduction to cell division. Cell division, or mitosis, is the process by which a mother cell divides its nuclear and cytoplasmic components into two daughter cells. Mitosis is divided into four major phases: prophase, metaphase, anaphase, and telophase. Careful regulation of the cell division program is crucial for proper cell growth ...
Aims and scope. Cell Division is an open access, peer-reviewed journal, which publishes articles in areas including, but not limited to, molecular aspects of cell cycle control and cancer, cell growth, proliferation, survival, differentiation, signalling, gene transcription, protein synthesis, genome integrity, chromosome stability, centrosome ...
Abstract. Cancer is a group of diseases in which cells divide continuously and excessively. Cell division is tightly regulated by multiple evolutionarily conserved cell cycle control mechanisms ...
Early studies seeking to dissect the mechanisms of cell division used classical genetics approaches to identify genes involved in mitosis and deployed biochemical approaches to isolate and identify proteins critical for cell division. These studies underscored that post-translational modifications and cyclin-kinase complexes play roles at the ...
Cell division, or mitosis, is the process. by which a mother cell divides its nuc lear and. cytoplasmic components into two daughter cells. Mitosis is divided into four major phases: prophase ...
The cellular growth and division cycle. (A) Cartoon of the main segments of the cell cycle.During interphase (G 1, S, G 2), the cell accomplishes sufficient biosynthesis to become two.In mitosis (M), cell parts are reorganized so the mitotic spindle can achieve the equipartition of the chromosomes and centrosomes, leaving the distribution of more numerous components, such as ribosomes, to the ...
The cell cycle. 'Dividing cells pass through a regular sequence of cell growth and division, known as the cell cycle', according to a college textbook of biology published in 1983 [ 1 ], 5 years before the underlying principles of control were first laid bare during 1988, the annus mirabilis of cell cycle research [ 2, 3 ].
Cell division requires a massive rewiring of cellular pathways, including molecular routes involved in providing energy for cell survival and functionality. The energetic requirements and the metabolic opportunities for generating energy change during the different phases of the cell cycle and how these processes are connected is still poorly ...
July 27, 2021 — New research has uncovered an essential mechanism coordinating the processes of cell division and adhesion within humans. This discovery has profound potential for advancing ...
Cell division. Jonathan M. Scholey, Ingrid Brust-Mascher & Alex Mogilner. Laboratory of Cell and Computational Biology, Center for Genetics and D evelopment, University of California, Davis ...
Read the latest Research articles in Cell division from Scientific Reports. ... Relating individual cell division events to single-cell ERK and Akt activity time courses. ... Calls for Papers
Cell Division provides an online forum for the cell-cycle community that aims to publish articles on all exciting aspects of cell-cycle research and to bridge the gap between models of cell cycle regulation, development, and cancer biology. This forum is driven by specialized and timely research articles, reviews and commentaries focused on ...
State of research into conceptions of cells and cell division Research into students´ conceptions of cells and cell division could be differentiated into two ... For the 'Clarification of science subject matter' we used historical research papers and recent leading scientific textbooks as major sources (e.g., Hooke, 1667; Schwann, 1839 ...
The cell cycle is controlled by numerous mechanisms ensuring correct cell division. This review will focus on these mechanisms, i.e. regulation of cyclin‐dependent kinases (CDK) by cyclins, CDK inhibitors and phosphorylating events. The quality checkpoints activated after DNA damage are also discussed. The complexity of the regulation of the ...
We include primary research papers and review articles that delve into the crosstalk between motors, microtubules and signaling molecules during division, as well as manuscripts that examine the mechanisms by which cells ensure the faithful segregation of materials during meiosis or mitosis. Additional studies investigate how signaling cascades ...
Department of Zoology, Visva-Bhatati, 731235, Santiniketan. Abstract Cell division, perhaps the most important is among the most difficult topics in biology to teach. With respect to. the ...
Indeed, most cancers are the result of inappropriate cell division, often stemming from aberrations in normal cell cycle regulation. Considerable research is directed to identifying alterations in ...
For successful asymmetric division, the spindle must be positioned near the cell cortex and oriented perpendicularly to the cell cortex. Considerable studies on asymmetric divisions have been performed in mitosis, highlighting the critical roles of centrosomes and interaction between aster microtubules and the cell cortex ( Knoblich 2010 ).
The paper describes how strings and clusters of cells form within the marrow to act as blood cell production factories. Location matters, even during normal blood cell production.
Cell Division strongly encourages that all datasets on which the conclusions of the paper rely should be available to readers. We encourage authors to ensure that their datasets are either deposited in publicly available repositories (where available and appropriate) or presented in the main manuscript or additional supporting files whenever possible.
In this paper, assembling all the centromeres from a human cell line has allowed the authors to perform a first analysis of genetic variation across human centromeres.
PNSB Palau Fellowship Award Recipients Discuss Studying abroad and internships | August 7, 2024 | EPFM Studio
Cell death is one of the most important mechanisms of maintaining homeostasis in our body. Ferroptosis and pyroptosis are forms of necrosis-like cell death. These cell death modalities play key roles in the pathophysiology of cancer, cardiovascular, neurological diseases, and other pathologies. Transition metals are abundant group of elements in all living organisms. This paper presents a ...